-
PDF
- Split View
-
Views
-
Cite
Cite
Yanmin Yang, Luojia Yang, Jiankang Sun, Marco L. Gruwel, Roxanne Deslauriers, Jian Ye, A modified protocol for retrograde cerebral perfusion: magnetic resonance spectroscopy in pigs, European Journal of Cardio-Thoracic Surgery, Volume 43, Issue 5, May 2013, Pages 1065–1071, https://doi.org/10.1093/ejcts/ezs505
- Share Icon Share
Abstract
Retrograde cerebral perfusion (RCP) has been employed to protect the brain during cardiovascular surgery, requiring temporary hypothermic circulatory arrest (HCA). However, the protocol used for RCP remains to be modified if prolonged HCA is expected. The aim of this study was to determine the efficacy of a modified protocol for this purpose.
After establishment of HCA at 15°C, 14 pigs were subjected to 90-min RCP using either the conventional protocol (i.e. alpha-stat strategy, 25-mmHg perfusion pressure and occluded inferior vena cava, Group I, n = 7) or the new protocol (i.e. pH-stat strategy, 40-mmHg perfusion pressure and unoccluded inferior vena cava, Group II, n = 7). After being rewarmed to 37°C, pigs were perfused for another 60 min. Phosphorus-31 magnetic resonance spectroscopy was used to track the changes of brain high-energy phosphates [i.e. adenosine triphosphate and phosphocreatine (PCr)] and intracellular pH (pHi). At the end, brain water content was measured.
During RCP, high-energy phosphates decreased in both groups, whereas adenosine triphosphate decreased much faster in Group I (10.4 ± 4.3 vs 30.4 ± 4.4% of the baseline, P = 0.007, 60-min RCP). After rewarming, the recovery of high-energy phosphates and pHi was much slower in Group I (PCr: 55.7 ± 9.1 vs 78.4 ± 5.1% of the baseline, P = 0.046; adenosine triphosphate: 26.6 ± 10.6 vs 64.8 ± 4.6% of the baseline, P = 0.007; pHi: 6.5 ± 0.4 vs 7.1 ± 0.1, P = 0.021 at 30-min normothermic perfusion after rewarming). Brain tissue water content was significantly higher in Group I (81.1 ± 0.4 vs 79.5 ± 0.4%, P = 0.016).
Application of the modified RCP protocol significantly improved cerebral energy conservation during HCA and accelerated energy recovery after rewarming.
INTRODUCTION
Hypothermic circulatory arrest (HCA) is frequently used during aortic surgery, which can still incur severe neurological complications due to prolonged deficiency of nutrients, oxygen (O2) and gradual massive accumulation of metabolic wastes. Two adjunctive strategies, i.e. selective antegrade and retrograde cerebral perfusion (ACP and RCP), have been proposed and used to prolong the safe duration of HCA.
Initial hypothesis and animal studies indicated that selective ACP can provide sufficient amount of blood into the brain without, if any, injurious effects, which leads to its wide application. Subsequent studies further suggested that ACP can even be safely applied under mild (28–30°C) to moderate (24–26°C) hypothermic arrest [1–3]. However, retrospective clinical studies gradually revealed that the outcome of ACP might not be as perfect as we previously anticipated because it failed to maximize prevention of significant neurological dysfunction [4, 5] due mainly to cerebral embolization during both CPB and ACP [6]. Patients of older ages, with prolonged operative time or with other significant comorbidities were more likely to develop it [4]. In addition, although ACP can be applied in the majority of patients, it may not be suitable or appropriate in those patients with acute aortic dissection involving arch vessels, significant carotid or cerebral vascular stenosis, significant carotid atherosclerosis or unusual anatomy of the arch vessels.
As a result, RCP as an adjunct to HCA, introduced by Ueda et al. in 1990 [7], still has its field of usage in clinic. However, clinical practice indicates that its efficacy in cerebral protection for prolonged HCA still seems to be limited, as long RCP time (>60 min) remains a major predictor for postoperative mortality and neurological morbidity [8]. Our previous study in pigs suggested that, compared with ACP, RCP did only provide very limited amount of blood into the brain (<10%) when conventional perfusion protocol, i.e. alpha-stat strategy, occlusion of the inferior vena cava (IVC) and 20–25-mmHg perfusion pressure, was employed [9]. To pursue better protective efficacy, we had tested several modification schemes, which suggested that: (i) different managements of IVC during RCP played an important role in determining the maximum safe retrograde perfusion pressure with occluded IVC during RCP leading to high intracranial pressure that inversely risked brain perfusion and potentially incurred brain damage [10], (ii) with an open IVC, continuous higher perfusion pressure at 40–45 mmHg, which effectively improved brain perfusion, could be safely used [11, 12] and (iii) with an open IVC and higher perfusion pressure, use of pH-stat strategy (i.e. maintenance of pH at 7.40 corrected to core temperature during hypothermia) could further increase brain perfusion and slow down O2 exhaustion during RCP relative to the use of alpha-stat strategy [13, 14]. As such, a new protocol based on combination of all these modifications is undergoing development for its future application in clinic.
The aim of this study was to assess the effects of combination of these schemes during RCP on brain energy metabolism under deep hypothermia (15°C). To achieve this goal, phosphorus-31 magnetic resonance spectroscopy (31P-MRS) was employed to assess regional cerebral energy metabolism during cardiopulmonary bypass (CPB) and RCP in pigs in vivo.
MATERIALS AND METHODS
Animal preparation
As described previously [10, 11, 13], all pigs (∼30 kg) received human care in compliance with the guidelines of the Canadian Council on Animal Care [15]. Preoperative anaesthesia was induced with midazolam, ketamine and atropine. Mechanical ventilation was used to maintain an optimal level of arterial blood PaCO2 (35–45 mmHg), PaO2 (100–150 mmHg) and pH (7.35–7.45). Anaesthesia was maintained with 1.5–2.0% isoflurane. A temperature probe was placed in the oesophagus to monitor the core temperature.
The chest was opened via a median sternotomy, and CPB was established with cannulation of the ascending aorta and the right atrium. The superior vena cava (SVC) was cannulated with a modified 14F retrograde cardioplegic catheter for establishment of RCP (large lumen) and monitoring of SVC pressure (small lumen). In addition, a small cannula was placed into the right internal jugular vein through the left internal mammary vein for continuous measurement of retrograde perfusion pressure in the internal jugular vein and also for the collection of venous blood sample. During retrograde perfusion, the perfusion pressure measured via the internal jugular vein was maintained at the designated levels by adjusting the pump flow. Right internal mammary artery was cannulated to monitor arterial pressure. Sodium bicarbonate was added to adjust the pH to 7.40, when necessary. The bypass circuit was designed to allow alternative shift between normal CPB and RCP.
Groups and protocol
Fourteen pigs were randomly divided into two groups. Pigs in Group I received an alpha-stat strategy with 25-mmHg perfusion pressure and occluded IVC during RCP, a protocol of which was commonly used in clinical practice. Pigs in Group II received modified schemes, including a pH-stat strategy with 40-mmHg perfusion pressure and unoccluded IVC during RCP.
The protocol (Table 1) for both experimental groups consisted of 30-min normothermic CPB (baseline at 37°C), ∼35-min cooling, 30-min hypothermic CPB (baseline at 15°C), 90-min HCA with RCP, 30-min hypothermic CPB (15°C), ∼40-min rewarming and 60-min normothermic CPB. Extension of the RCP and reperfusion time with CPB following RCP was to maximize the effects of RCP with different protocols and changes following RCP. Observation of energy exhaustion kinetics during the period of RCP and reperfusion was an important task of this study. Application of pH-stat strategy was started at the beginning of cooling, an approach proved to be more efficient in improving cerebral blood flow than application of it at the beginning of RCP (unpublished data). During the whole process of CPB and RCP, all pigs underwent measurement of localized 31P-MRS. Arterial and venous blood gases were measured at each stage of the protocol to monitor blood gas and oxygen consumption. At the end of the experiments, the pig brain was removed immediately for quantitative measurements of tissue water content.
Bypass stage . | Normothermic CPB . | Hypothermic CPB . | RCP . | Hypothermic CPB . | Rewarming . | Normothermic CPB . | |
---|---|---|---|---|---|---|---|
Temperature (°C) | 37 | 37 → 15 | 15 | 15 | 15 | 15 → 37 | 37 |
Time (min) | 30 | ∼35 | 30 | 90 | 30 | ∼40 | 60 |
PP (mmHg) | |||||||
Group I | 60 | 60 | 60 | 25 | 60 | 60 | 60 |
Group II | 40 | ||||||
Acid-base strategy | |||||||
Group I | alpha-stat | alpha-stat | alpha-stat | alpha-stat | alpha-stat | alpha-stat | alpha-stat |
Group II | pH-stat | pH-stat | pH-stat | ||||
Inferior vena cava | |||||||
Group I | Open | Open | Open | Occlusion | Open | Open | Open |
Group II | Open | ||||||
Major measurement | 31P-MRS | 31P-MRS | 31P-MRS(x3) | 31P-MRS | 31P-MRS(x2) |
Bypass stage . | Normothermic CPB . | Hypothermic CPB . | RCP . | Hypothermic CPB . | Rewarming . | Normothermic CPB . | |
---|---|---|---|---|---|---|---|
Temperature (°C) | 37 | 37 → 15 | 15 | 15 | 15 | 15 → 37 | 37 |
Time (min) | 30 | ∼35 | 30 | 90 | 30 | ∼40 | 60 |
PP (mmHg) | |||||||
Group I | 60 | 60 | 60 | 25 | 60 | 60 | 60 |
Group II | 40 | ||||||
Acid-base strategy | |||||||
Group I | alpha-stat | alpha-stat | alpha-stat | alpha-stat | alpha-stat | alpha-stat | alpha-stat |
Group II | pH-stat | pH-stat | pH-stat | ||||
Inferior vena cava | |||||||
Group I | Open | Open | Open | Occlusion | Open | Open | Open |
Group II | Open | ||||||
Major measurement | 31P-MRS | 31P-MRS | 31P-MRS(x3) | 31P-MRS | 31P-MRS(x2) |
The grouping was based on perfusion pressure during RCP, acid-base strategy and different management of IVC. 31P-MRS: phosphorus-31 magnetic resonance spectroscopy; CPB: cardiopulmonary bypass; PP: perfusion pressure; RCP: retrograde cerebral perfusion; IVC: inferior vena cava. The bold/bold-italic terms indicate the differences in the experimental protocol between the two groups.
Bypass stage . | Normothermic CPB . | Hypothermic CPB . | RCP . | Hypothermic CPB . | Rewarming . | Normothermic CPB . | |
---|---|---|---|---|---|---|---|
Temperature (°C) | 37 | 37 → 15 | 15 | 15 | 15 | 15 → 37 | 37 |
Time (min) | 30 | ∼35 | 30 | 90 | 30 | ∼40 | 60 |
PP (mmHg) | |||||||
Group I | 60 | 60 | 60 | 25 | 60 | 60 | 60 |
Group II | 40 | ||||||
Acid-base strategy | |||||||
Group I | alpha-stat | alpha-stat | alpha-stat | alpha-stat | alpha-stat | alpha-stat | alpha-stat |
Group II | pH-stat | pH-stat | pH-stat | ||||
Inferior vena cava | |||||||
Group I | Open | Open | Open | Occlusion | Open | Open | Open |
Group II | Open | ||||||
Major measurement | 31P-MRS | 31P-MRS | 31P-MRS(x3) | 31P-MRS | 31P-MRS(x2) |
Bypass stage . | Normothermic CPB . | Hypothermic CPB . | RCP . | Hypothermic CPB . | Rewarming . | Normothermic CPB . | |
---|---|---|---|---|---|---|---|
Temperature (°C) | 37 | 37 → 15 | 15 | 15 | 15 | 15 → 37 | 37 |
Time (min) | 30 | ∼35 | 30 | 90 | 30 | ∼40 | 60 |
PP (mmHg) | |||||||
Group I | 60 | 60 | 60 | 25 | 60 | 60 | 60 |
Group II | 40 | ||||||
Acid-base strategy | |||||||
Group I | alpha-stat | alpha-stat | alpha-stat | alpha-stat | alpha-stat | alpha-stat | alpha-stat |
Group II | pH-stat | pH-stat | pH-stat | ||||
Inferior vena cava | |||||||
Group I | Open | Open | Open | Occlusion | Open | Open | Open |
Group II | Open | ||||||
Major measurement | 31P-MRS | 31P-MRS | 31P-MRS(x3) | 31P-MRS | 31P-MRS(x2) |
The grouping was based on perfusion pressure during RCP, acid-base strategy and different management of IVC. 31P-MRS: phosphorus-31 magnetic resonance spectroscopy; CPB: cardiopulmonary bypass; PP: perfusion pressure; RCP: retrograde cerebral perfusion; IVC: inferior vena cava. The bold/bold-italic terms indicate the differences in the experimental protocol between the two groups.
Phosphorus-31 magnetic resonance spectroscopy
The process of 31P-MRS was detailed in our previous studies [16]. Briefly, data were acquired with a Bruker Biospec spectrometer (Bruker Medical Instruments, Inc., Billerica, MA, USA) equipped with a Magnex 7T/40-cm magnet and actively shielded gradients with a clear bore of 30 cm. After completion of the operation, the pig was placed in the supine position in a polyvinylchloride cradle with its head secured to a 1H-RF coil (300.1 MHz) attached to the cradle. The pig was then positioned with its head at the magnet isocenter and a transverse scout MR image was acquired. From this scout image, a 2-cm thick coronal slice through the posterior part of the brain was selected and imaged (spin echo, TR = 1000 ms, TE = 20 ms, 5 mm thick and field of view = 16 cm).
After exchange of the 1H-RF coils to a 31P-RF coil (121.5 MHz), calibration of RF power was then performed by collecting the 31p signal from the entire selected 2-cm coronal slice and adjusting the power to maximize the high-energy phosphate signal. A 2D spectroscopic image dataset was acquired with a gradient echo sequence (TE = 1.5 ms, TR = 1500 ms and field of view = 16 cm), with two phase-encoding gradients applied in-plane with the selected slice (with eight increments along each axis for a nominal voxel size of 8 cm3), spectral width = 10 kHz, 2048 acquisition points, 1-ms gradient pulses with a 0.5-ms rise-time and 1-ms slice-selective Gaussian RF pulse for a total acquisition time of 25.6 min. The timing of each acquisition during the experiment was detailed in Table 1.
The acquired dataset was then processed with the UXNMR (Bruker). For quantification of peak areas, the unphased complex spectra were fitted with in-house software (ALLFIT). Fitted metabolite peak areas and chemical shifts were tabulated, and the total adenosine triphosphate (i.e. α + β + γ ATP peak), phosphocreatine (PCr) and inorganic phosphate (Pi) levels were determined. Intracellular pH (pHi) was calculated from the chemical shift difference between Pi and PCr, taking into account the variation in temperature throughout the experiment.
Brain tissue water content
Blocks of tissue from different regions in the brains were obtained and weighed. After weighing, the tissue was dried at 60°C and weighed daily until a constant weight was obtained (typically 72 h). Tissue water content (%) = (wet weight – dry weight)/(wet weight) × 100%.
Statistical analysis
The data were expressed as the mean ± standard error of the deviation. Statistics were performed using statistical software (Statistica 6.0, Statsoft Inc., Tulsa, OK, USA). All the spectroscopic data except pHi were normalized to their respective baseline values measured at the beginning of CPB and expressed as a percentage of the baseline values. Repeated measures analysis of variance and Duncan's multiple range tests were used for comparison between different time points within a group, and the Student's t-test was used for comparison between the two groups. A P < 0.05 was considered significant.
RESULTS
There were no significant differences in the pigs' age (14.4 ± 0.2 weeks in Group I vs 14.5 ± 0.3 weeks in Group II) and body weight (30.4 ± 1.0 kg in Group I vs 31.5 ± 1.5 kg in Group II). Table 2 showed recorded key perfusion parameters, which strictly followed the initial protocol design. To maintain a higher perfusion pressure, pump flow was higher in Group II during RCP (4.1 ± 0.4 ml kg−1 min−1 in Group I vs 15.2 ± 2.0 ml kg−1 min−1 in Group II, P < 0.001).
Body temperature, cerebral perfusion pressure and pump flow during experiments
Bypass stage . | Normothermic CPB . | Hypothermic CPB after cooling . | RCP . | Hypothermic CPB before rewarming . | Normothermic CPB after rewarming . |
---|---|---|---|---|---|
Temperature (°C) | |||||
Group I | 37.0 ± 0.6 | 15.1 ± 0.1 | 15.3 ± 0.5 | 15.1 ± 0.2 | 36.8 ± 0.3 |
Group II | 36.9 ± 0.8 | 15.0 ± 0.2 | 15.1 ± 0.3 | 15.2 ± 0.3 | 37.0 ± 0.2 |
Perfusion pressure (mmHg) | |||||
Group I | 59.6 ± 1.2 | 60.2 ± 0.7 | 24.5 ± 0.5 | 59.9 ± 1.1 | 60.3 ± 0.6 |
Group II | 60.1 ± 0.8 | 60.3 ± 1.2 | 40.6 ± 0.6* | 60.5 ± 1.5 | 59.8 ± 1.1 |
Pump flow (ml kg−1 min−1) | |||||
Group I | 90.6 ± 3.6 | 89.1 ± 4.2 | 4.1 ± 0.4 | 91.1 ± 5.2 | 88.9 ± 4.1 |
Group II | 88.2 ± 4.5 | 91.7 ± 3.9 | 15.2 ± 2.0* | 89.3 ± 5.7 | 91.2 ± 4.9 |
Bypass stage . | Normothermic CPB . | Hypothermic CPB after cooling . | RCP . | Hypothermic CPB before rewarming . | Normothermic CPB after rewarming . |
---|---|---|---|---|---|
Temperature (°C) | |||||
Group I | 37.0 ± 0.6 | 15.1 ± 0.1 | 15.3 ± 0.5 | 15.1 ± 0.2 | 36.8 ± 0.3 |
Group II | 36.9 ± 0.8 | 15.0 ± 0.2 | 15.1 ± 0.3 | 15.2 ± 0.3 | 37.0 ± 0.2 |
Perfusion pressure (mmHg) | |||||
Group I | 59.6 ± 1.2 | 60.2 ± 0.7 | 24.5 ± 0.5 | 59.9 ± 1.1 | 60.3 ± 0.6 |
Group II | 60.1 ± 0.8 | 60.3 ± 1.2 | 40.6 ± 0.6* | 60.5 ± 1.5 | 59.8 ± 1.1 |
Pump flow (ml kg−1 min−1) | |||||
Group I | 90.6 ± 3.6 | 89.1 ± 4.2 | 4.1 ± 0.4 | 91.1 ± 5.2 | 88.9 ± 4.1 |
Group II | 88.2 ± 4.5 | 91.7 ± 3.9 | 15.2 ± 2.0* | 89.3 ± 5.7 | 91.2 ± 4.9 |
CPB: cardiopulmonary bypass; RCP: retrograde cerebral perfusion.
*P < 0.01 vs Group I.
Body temperature, cerebral perfusion pressure and pump flow during experiments
Bypass stage . | Normothermic CPB . | Hypothermic CPB after cooling . | RCP . | Hypothermic CPB before rewarming . | Normothermic CPB after rewarming . |
---|---|---|---|---|---|
Temperature (°C) | |||||
Group I | 37.0 ± 0.6 | 15.1 ± 0.1 | 15.3 ± 0.5 | 15.1 ± 0.2 | 36.8 ± 0.3 |
Group II | 36.9 ± 0.8 | 15.0 ± 0.2 | 15.1 ± 0.3 | 15.2 ± 0.3 | 37.0 ± 0.2 |
Perfusion pressure (mmHg) | |||||
Group I | 59.6 ± 1.2 | 60.2 ± 0.7 | 24.5 ± 0.5 | 59.9 ± 1.1 | 60.3 ± 0.6 |
Group II | 60.1 ± 0.8 | 60.3 ± 1.2 | 40.6 ± 0.6* | 60.5 ± 1.5 | 59.8 ± 1.1 |
Pump flow (ml kg−1 min−1) | |||||
Group I | 90.6 ± 3.6 | 89.1 ± 4.2 | 4.1 ± 0.4 | 91.1 ± 5.2 | 88.9 ± 4.1 |
Group II | 88.2 ± 4.5 | 91.7 ± 3.9 | 15.2 ± 2.0* | 89.3 ± 5.7 | 91.2 ± 4.9 |
Bypass stage . | Normothermic CPB . | Hypothermic CPB after cooling . | RCP . | Hypothermic CPB before rewarming . | Normothermic CPB after rewarming . |
---|---|---|---|---|---|
Temperature (°C) | |||||
Group I | 37.0 ± 0.6 | 15.1 ± 0.1 | 15.3 ± 0.5 | 15.1 ± 0.2 | 36.8 ± 0.3 |
Group II | 36.9 ± 0.8 | 15.0 ± 0.2 | 15.1 ± 0.3 | 15.2 ± 0.3 | 37.0 ± 0.2 |
Perfusion pressure (mmHg) | |||||
Group I | 59.6 ± 1.2 | 60.2 ± 0.7 | 24.5 ± 0.5 | 59.9 ± 1.1 | 60.3 ± 0.6 |
Group II | 60.1 ± 0.8 | 60.3 ± 1.2 | 40.6 ± 0.6* | 60.5 ± 1.5 | 59.8 ± 1.1 |
Pump flow (ml kg−1 min−1) | |||||
Group I | 90.6 ± 3.6 | 89.1 ± 4.2 | 4.1 ± 0.4 | 91.1 ± 5.2 | 88.9 ± 4.1 |
Group II | 88.2 ± 4.5 | 91.7 ± 3.9 | 15.2 ± 2.0* | 89.3 ± 5.7 | 91.2 ± 4.9 |
CPB: cardiopulmonary bypass; RCP: retrograde cerebral perfusion.
*P < 0.01 vs Group I.
Brain intracellular pH
The value of pHi measured in pig brain tissue at an interval of 30 min during initial CPB (normothermic and 15°C CPB), RCP (30, 60 and 90 min), reperfusion (15°C CPB) and after completion of rewarming (30-and 60-min normothermic CPB) were detailed in Fig. 1. During the period of normothermic and 15°C bypass, pHi remained normal (∼7.0) in either groups. During RCP, pHi in each group became gradually acidic with no difference between groups. However, recovery of pHi after reperfusion was significantly faster in Group II than in Group I (6.2 ± 0.2 in Group I vs 6.9 ± 0.1 in Group II at 30-min normothermic CPB after rewarming, P = 0.049).
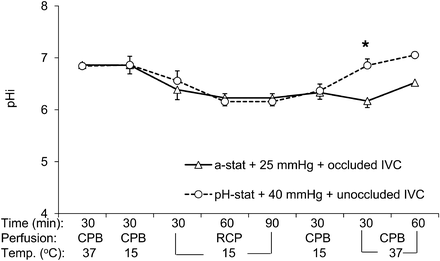
Changes in brain intracellular pH (pHi) determined by phosphorus-31 magnetic resonance spectroscopy during RCP with conventional protocol (alpha-stat strategy, 25-mmHg perfusion pressure and occluded IVC, triangles) or modified perfusion condition (i.e. pH-stat strategy, 40-mmHg perfusion pressure and open IVC, circles) groups. CPB: cardiopulmonary bypass; IVC: inferior vena cava; RCP: retrograde cerebral perfusion; Temp.: temperature (*P < 0.05 vs conventional perfusion group).
Brain high-energy phosphates
Representative 31P-MRS spectra changes in relation to different perfusion were shown in Fig. 2. As indicated in Fig. 3, PCr did not experience any decrement after hypothermic bypass in both groups. During the period of RCP at 15°C, PCr in both groups decreased progressively with no statistical difference between groups. During reperfusion at 15°C, recovery of PCr tended to be faster in Group II. Thirty minutes after rewarming, PCr had recovered above 70% of the baseline level in Group II, whereas it was just over 50% in Group I (55.7 ± 9.1% in Group I vs 78.4 ± 5.1% of the baseline in Group II, 30-min normothermic CPB, P = 0.046).
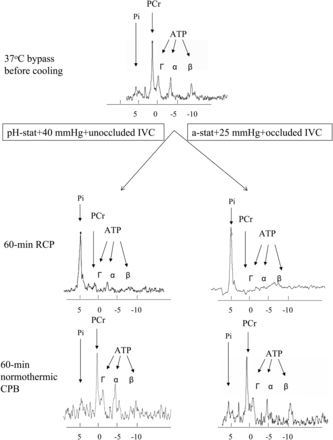
Representative MR spectra from the two groups: right, retrograde cerebral perfusion with conventional protocol (alpha-stat, 25-mmHg perfusion pressure, occluded inferior vena cava); left, retrograde cerebral perfusion with modified protocol (pH-stat, 40-mmHg perfusion pressure and open inferior vena cava).
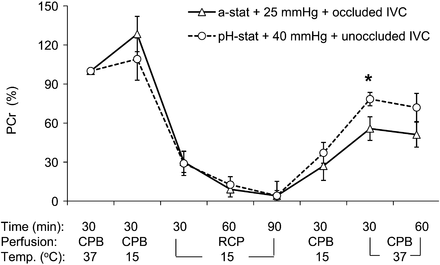
Changes in brain phosphocreatine (PCr) determined by phosphorus-31 magnetic resonance spectroscopy during RCP with conventional protocol (alpha-stat strategy, 25-mmHg perfusion pressure and occluded IVC, triangles) or modified perfusion condition (i.e. pH-stat strategy, 40-mmHg perfusion pressure and open IVC, circles) groups. CPB: cardiopulmonary bypass; IVC: inferior vena cava; RCP: retrograde cerebral perfusion; Temp.: temperature (*P < 0.05 vs conventional perfusion group).
Figure 4 showed respective changes of ATP level in both groups during the process of CPB and RCP. After hypothermic CPB, brain ATP level in both groups remained unchanged when compared with its original normothermic level. However, during the subsequent 90-min RCP, ATP decreased progressively with a faster decay rate in Group I than in Group II (10.4 ± 4.3 vs 30.4 ± 4.4% of the baseline, 60-min RCP, P = 0.007). At the end of RCP, ATP in the brain was almost completely depleted in both groups. During reperfusion at 15°C, recovery of ATP was significantly faster in Group II than in Group I. After the first 30-min normothermic bypass following rewarming, ATP had recovered to 64.8 ± 4.6% in Group II when compared with it only to 26.6 ± 10.8% in Group I (P = 0.007). At the end of normothermic bypass, ATP had recovered to 81.3 ± 13.7% in Group II when compared with only 32.1 ± 12.5% of the baseline level in Group I (P = 0.021).
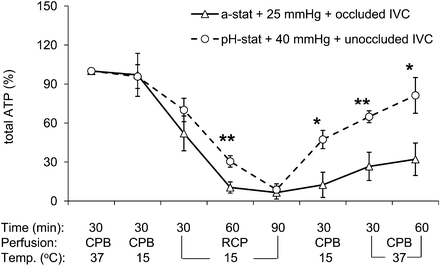
Changes in brain total ATP (α + β + γ) determined by phosphorus-31 magnetic resonance spectroscopy during RCP with conventional protocol (alpha-stat strategy, 25-mmHg perfusion pressure and occluded IVC, triangles) or modified perfusion condition (i.e. pH-stat strategy, 40-mmHg perfusion pressure and open IVC, circles) groups. RCP: retrograde cerebral perfusion; Temp.: temperature; IVC: inferior vena cava. (*P < 0.05, **P < 0.01 vs conventional perfusion group).
Brain tissue water content
At the end of bypass, the brain tissue accumulated significantly higher amount of water in Group I than in Group II (81.1 ± 0.4 vs 79.5 ± 0.4%, P = 0.016). The water content in Group II was similar to that in the normal control pigs (78.7 ± 0.2%) reported in our previous publication [11].
DISCUSSION
Although hypothermia can significantly decrease the metabolic rate of the brain, it cannot completely shut off metabolism even under deep hypothermia (<20°C) [17]. Yager et al. [18] found that after onset of HCA at 18°C in dogs, glucose decreased progressively in cerebral cortex, caudate nucleus, hippocampus and subcortical white matter to negligible levels by 30 min. As a result, during cardiovascular surgery requiring prolonged HCA, provision of adjunct flow into the brain to prevent ischaemic injury is highly desirable.
In clinic, newly developed brain damage after cardiovascular surgery has been categorized into either permanent neurological deficit or transient brain dysfunction. Transient brain dysfunction is usually a functional change and believed to be a reflection of gross reversible ischaemia of the whole brain, whereas, permanent neurological deficit is usually related to cerebral embolization (air or debris) developed during perfusion and detected by CT scan or clinical presentation postoperatively. Selective ACP is currently a preferred choice as it can reduce the incidence of transient brain dysfunction due to uniform and sufficient provision of blood flow to the brain [19, 20]. However, occurrence of permanent neurological deficits remained a concern, especially, in patients of older age, with advanced vessel diseases requiring extensive manipulation or with multiple comorbidities [21]. Also, if patients suffered from acute aortic dissection involving arch vessels, significant carotid or cerebral vascular stenosis, significant carotid atherosclerosis or other unusual anatomy of the arch vessels, ACP may not be suitable or appropriate and RCP would be a better alternative choice. In addition, RCP has been the choice of the treatment for accidental massive air embolization during CPB.
Although RCP remains a choice for some specific cardiovascular procedures, the protocol currently used in clinic has not consistently demonstrated that RCP provides sufficient blood to support cerebral metabolism and prevent cerebral ischaemia during HCA [9, 22]. Our recent serial studies have respectively indicated that unocclusion of IVC, higher perfusion pressure at 40–50 mmHg, or pH-stat strategy could improve brain tissue perfusion and oxygenation during RCP [10, 11, 14]. Among these modifications, continuous higher perfusion pressure during RCP seems to be especially optimistic as its pilot use as an intermittent scheme (cyclic 30-s high perfusion pressure followed by 120-s routine perfusion pressure during RCP) in patients has been observed to be efficient in reducing the rate of brain O2 depletion during RCP and accelerating wakening after surgery [23]. This study further suggested that the new protocol based on combination of these schemes did significantly slow down the decay rate of ATP and accelerate recovery of the high-energy phosphates (ATP and PCr) and pHi in brain.
As what we have suggested in our previous study, the reason for significantly prolonged retention of ATP during RCP was related to improved brain perfusion during the stage of both hypothermic CPB and RCP. During the initial stage of hypothermic CPB, initiation of pH-stat strategy can (i) improve the homogeneity of brain cooling before institution of HCA [24] due to significantly enhanced blood flow [14] and prevention of blood flow redistribution [25], (ii) enhance O2 delivery due to partial correction of left-shifted O2-hemoglobin dissociation curve during hypothermia and (iii) provide more oxygenated blood reserve (∼20%) at the beginning of RCP [13, 14]. During RCP, both higher tissue perfusion and pH-stat strategy contributed to delayed depletion of ATP in Group II probably because (i) more nutrients and O2 were delivered to the brain and (ii) tissue temperature was controlled more efficiently. Another interesting finding in this study was that, after RCP, recovery of ATP was also much faster using the new protocol. Within 1 h of normothermic reperfusion, >80% ATP recovered in Group II when compared with 1/3 ATP in Group I. As what has been indicated in our previous experiment, possible explanation for the improved energy recovery included both reduced ischaemic injury during RCP and improved tissue perfusion and oxygenation during reperfusion [13, 14].
However, the new protocol still could not delay the decay of PCr during RCP. It is well known that PCr is a phosphorylated creatine molecule that is an important energy store in the brain. It is used to anaerobically generate ATP from ADP by donating a phosphate group via catalysis of creatine kinase. As a result, the lack of sufficient blood flow during RCP will inevitably lead to exhaustion of first-line intrinsic energy reservoir, PCr, to immediately compensate for the net loss of ATP with the time elapsing. However, like ATP, the recovery of PCr was significantly slower, and only ∼50% was restored in Group I after 1-h normothermic reperfusion.
Another major finding in this study was that pHi inevitably became more acidic in both groups during RCP, but its recovery was quicker and more complete in Group II as compared to that in Group I. With the progression of RCP with either management, pHi turned acidic due to the gradual loss of high-energy phosphate, though the rate of such a loss was slower in Group II. The reason for this acidic shift was due to accumulation of metabolic intermediates (e.g. lactate) resulting from (i) the change from aerobic to anaerobic metabolism due to more (in Group I) or less (in Group II) deficiency in O2 and carbohydrates and (ii) the lack of blood flow to flush out these intermediates. During reperfusion, pHi quickly returned to its baseline level in Group II due possibly to significantly higher tissue perfusion [13, 14] leading to (i) faster restoration of nutrients supply and thus aerobic metabolism, as well as (ii) faster removal of acidic intermediates out of neurons when compared with retarded restoration of tissue perfusion in Group I.
A major concern related to the use of pH-stat strategy during hypothermic CPB is potentially increased possibility of brain embolic injury and decreased pH that may suppress enzyme activity. However, RCP, by itself, is able to flush out cerebral emboli, and suppression of enzyme activity may not be the case as the use of the new protocol leads to a faster and more complete recovery of high-energy phosphate.
This study has some limitations. As a result of anatomical differences between humans and animals such as more competent valve in the human SVC as well as preoperative difference of pathophysiological state between human and animals (significantly higher occurrence of comorbidities in human beings such as diabetes and hypertension), our management may need further refinements for its subsequent translation in clinic. However, animal models provide a well-controlled experimental condition and allow measurements that often are not feasible in humans.
In conclusion, this study provided an added proof that the modified protocol could both prolong the safe time of RCP during HCA and accelerate the recovery of brain energy metabolism after reperfusion. Therefore, to improve the efficacy of brain protection during RCP, it may be desirable to employ the new protocol, i.e. pH-stat strategy, unclamped IVC and 40-mmHg perfusion pressure.
Funding
This work was supported in part by the Canadian Institutes of Health Research (grant numbers: 15352 and 42671 to J. Ye).
Conflict of interest: none declared.
ACKNOWLEDGEMENTS
The authors thank Allan Turner, Lori Gregorash, Shelly Germsheid, Rachelle Mariash and Denis Olynick for assistance in experiments.
REFERENCES
- polymerase chain reaction
- rewarming
- hypothermia, natural
- adenosine triphosphate
- cardiovascular surgical procedures
- objective (goal)
- magnetic resonance spectroscopy
- perfusion
- phosphates
- phosphocreatine
- suidae
- inferior vena cava
- brain
- phosphorus
- intracellular ph
- brain tissue
- normothermia
- cerebral perfusion
- thermodynamic energy conservation
- circulatory arrest
- prostate health index