-
PDF
- Split View
-
Views
-
Cite
Cite
Staffan Svenmarker, Sören Häggmark, Magnus Hultin, Anders Holmgren, Static blood-flow control during cardiopulmonary bypass is a compromise of oxygen delivery, European Journal of Cardio-Thoracic Surgery, Volume 37, Issue 1, January 2010, Pages 218–222, https://doi.org/10.1016/j.ejcts.2009.05.019
- Share Icon Share
Abstract
Background: Blood-flow control during cardiopulmonary bypass (CPB) is by tradition based on the patient’s body surface area. Emergence of new techniques enables dynamic blood-flow control based on online measurement of venous oxygen saturation and oxygen consumption. Present investigation aimed to compare static versus dynamic blood-flow control with respect to use of oxygen and effects upon organ function. Methods: In this study, 100 coronary-artery-bypass surgical patients were prospectively randomised to static or dynamic hypothermic blood-flow control during CPB. In the static group, pump flow was set to 2.4 (litres per minute) times the patient’s body surface area (m2) throughout the procedure. Pump flow in the dynamic group was varied according to the reading of the venous oxygen saturation and maintained at >75%. CPB-specific information was collected online. Blood samples were collected for analysis of haemoglobin, lactate, amylase, creatinine and C-reactive protein: pre-CPB, at weaning from CPB and on day 1 postoperatively. Results: Randomisation formed two uniform groups. Choice of static or dynamic blood-flow control during CPB had no significant effects on organ function as judged by lactate, amylase or creatinine levels. On increasing oxygen demand, oxygen balance was maintained by increasing venous oxygen extraction rates in the static flow mode and by increasing the pump flow rate in the dynamic group. Conclusions: Independent of the blood-flow control mode, oxygen balance remained preserved. However, the dynamic mode provided higher oxygen delivery, which may increase margins of safety and protection of organ function.
1 Introduction
Blood-flow control during cardiopulmonary bypass (CPB) is by tradition based on simple arithmetic using the patient’s body surface area (BSA) as reference [1,2]. Despite this very crude method of determining what represents adequate blood flow during CPB, it still remains the gold standard – more than 50 years since the method was first described by Kirklin et al. in 1957 [3]. The use of CPB is, for a variety of reasons, a dynamic process, with substantial fluctuations in blood-flow requirements related to the use of hypothermia, anaesthesia, haemodilution and overall metabolic demands [4,5]. The use of static blood flow per CPB matched for patient size or BSA may therefore seem unjustified from a physiological viewpoint [6].
Online monitoring during CPB with direct information of the patient’s circulatory and metabolic status [7] enables direct intervention and control of pump rate to meet actual blood-flow requirements. Oxidative phosphorylisation and adenosine triphosphate generation – fundaments of normal metabolism and tissue oxygenation – rely on functional balance between oxygen delivery and demand. Oxygen delivery during CPB is primarily controlled by the pump rate, with actual oxygen-carrying capacity as the essential co-factor [8], while variations in oxygen demand is often revealed by the oxygen extraction rate, equal to the converted actual venous oxygen saturation reading [9]. Dynamic pump rate adjustments to parallel metabolic needs based on the mixed venous oxygen saturation offers an alternative approach of blood-flow control during CPB compared to conventional accepted standards.
The present study aims to compare static versus dynamic blood-flow control during CPB in a series of coronary bypass surgery patients, with reference to global oxygenation and organ function.
2 Material and methods
2.1 Randomisation
The study included 100 first-time patients scheduled for aorto-coronary bypass surgery after informed consent and institutional ethical approval, randomised into two groups based on principles of blood-flow control during CPB. Randomisation was blinded for surgeon, anaesthesiologist and personnel responsible for postoperative care. Patients older than 80 years and those having diabetes mellitus were not included.
In the static group (n = 50), pump rate was calculated in litres per minute as the patient’s BSA times a factor of 2.4 and maintained constant throughout the procedure. In the dynamic group (n = 50), pump rate was synchronised to maintain the mixed venous oxygen saturation greater than 75%. For both the groups, the mean arterial pressure was kept above 50 mmHg using either phenylephrine or norepinephrine, as indicated. Arterial oxygen partial pressure was measured online (Data Master, Sorin Group, Mirandola, Italy) and maintained at 20 kPa.
2.2 Anaesthesia
The patients were anaesthetised in a similar fashion using morphine for pre-medication, propofol for induction and fentanyl–pancuronium combined with isoflurane in oxygen/air mixture for maintenance. Normoventilation and adequate oxygenation was the objective. Upon weaning from CPB, vasoactive and inotrope support was used if needed.
2.3 Control of cardiopulmonary bypass
Hypothermic (34 °C) CPB was instituted following successful anticoagulation with heparin (≥350 IU kg−1) confirmed by the activated clotting time greater than 480 s. Venous blood drained from the right atrium through a two-stage cannula was returned to the ascending aorta using the Stöckert S5 roller pump (Sorin Group Munich, Germany) and non-pulsatile membrane oxygenation using Trillium Affinity NT (Medtronic Inc., MN, USA) or Quadrox (Maquet Ltd., Rastatt, Germany). The CPB circuit was primed with 1000 ml Ringer’s acetate, 400 ml mannitol, 10 000 IU heparin and 80 mmol sodium chloride. Acid base management was according to alpha stat. A cardiotomy suction was used while no arterial inline filtration was employed. Myocardial protection was achieved by intermittent antegrade crystalloid cardioplegia. On completion of the last distal anastomoses, re-warming was begun with 37 °C in nasopharynx as target, the aortic clamp was removed and the proximal anastomoses were completed behind a side-biting clamp.
2.4 Data management and calculations
Online monitoring of circulatory and metabolic indices from the heart–lung machine; anaesthesia and inline monitors (Data Master, Sorin Group, Mirandola, Italy) was performed using Stöckert Data Management System (DMS). The gathered information was stored into a database and updated once every minute. The following were computed: arterial oxygen partial pressure (PaO2), mixed venous oxygen saturation (SvO2), pump rate (PR), mean arterial pressure (MAP), systemic vascular resistance (SVR), body surface area (BSA), haemoglobin (Hb), arterial oxygen content (CaO2), systemic oxygen delivery (DO2) and systemic oxygen consumption (VO2) based on the following calculations:
PR (l min−1) = BSA × 2.4.
SVR (dyn s cm−5) = (MAP/PR) × 80.
PRI (l min−1 m−2) = PR/BSA.
CaO2 (ml l−1) = (Haemoglobin × 1.39 × SaO2) + 0.031 × PaO2.
DO2 (ml min−1) = PR × CaO2.
VO2 (ml min−1) = DO2 × SvO2 × 10−3.
The indoor hospital database was used to evaluate patient perioperative course.
2.5 Blood sampling and analysis
Venous blood was collected before induction of anaesthesia (baseline), upon weaning from bypass (CPB) and in the intensive care unit (ICU) on postoperative day 1 for analysis of haemoglobin, lactate, amylase, creatinine and C-reactive protein. Lactate was analysed at bedside using ABL 725 (Radiometer Medical ApS, Denmark), whereas the remaining analyses were made by the chemistry laboratory in our hospital. Results were corrected for haemodilution using the red-cell volume fraction as reference.
2.6 Statistical analysis
Data collection was 99% complete. Categorical data was cross-tabulated and statistical inference was analysed using either the chi-square or Fischer’s exact test, whenever appropriate. Continuous data fulfilling an approximate normal distribution was analysed using Student’s t-test; otherwise, non-parametric techniques were employed, preferably the Mann–Whitney U-test. Repeated-measures analysis of variance was implemented if analysis of more than two data points were required and expressed as group difference and interaction effects. The P-values for individual observations were given in cases where statistically significant overall group differences were identified. Data are presented as means ± the standard error of the mean (SEM), if not otherwise stated. A P-value less than 0.05 was considered as statistically significant. Data were analysed using SPSS statistical package version 14.0.
3 Results
Two patients were excluded from the study; in one case because of excessive capillary leakage during CPB and in another due to randomisation error. The remaining 98 patients (50 + 48) formed two uniform groups as presented in Table 1 .
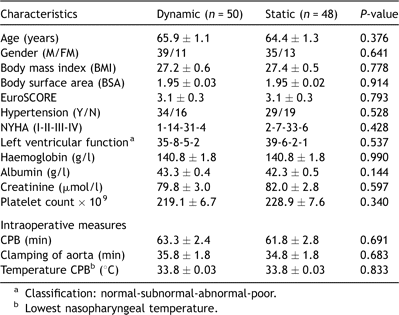
The mean arterial blood pressure varied on the average from 56.6 ± 0.8 to 62.3 ± 1.1 mmHg during the course of CPB, without any differences between the groups (P = 0.432). Systemic vascular resistance was throughout and significantly lower in the dynamic group (P = 0.000), ranging from 720 ± 21.1 to 888 ± 28.0 dyn s cm−5 and in the static group from 872 ± 28.4 to 991 ± 32.2 dyn s cm−5, respectively. Oxygen consumption was calculated to approximately 125 ml min−1 at stable hypothermia and increased significantly (P = 0.000) to ≈170 ml min−1 upon re-warming (T4). The increase was unrelated to the use of static or dynamic blood-flow control (P = 0.611). On increasing oxygen demand (T4), oxygen balance was maintained by increasing the pump rate index (P = 0.000) and thereby also the systemic oxygen delivery (P = 0.000) in the dynamic group and in the static group by increasing the venous oxygen extraction rate, seen as a significantly lower (P = 0.000) mixed venous oxygen saturation (Fig. 1 ).
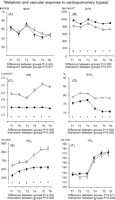
Online registration of metabolic and vascular response to CPB encompassing: Mean arterial pressure (MAP), systemic vascular resistance (SVR), pump rate index (PRI), mixed venous oxygen saturation (SvO2) systemic oxygen delivery (DO2) and systemic oxygen consumption (VO2). T1 = 10 min after start of CPB, T2 = 20 min after start of CPB, T3 = 5 min before re-warming, T4 = 10 min after re-warming, T5 = 20 min after re-warming, T6 = 5 min before weaning from CPB. Statistical results refer to analysis of variance (ANOVA), repeated measures. †P ≪ 0.05, ‡P ≪ 0.01, *P ≪ 0.001. (-○-) Dynamic group; (-●-) static group.
The lactate level increased (P = 0.000) after commencement of CPB and declined (P = 0.000) postoperatively, without any differences between the groups (P = 0.432). The creatinine level rose (P = 0.000) during CPB and continued to rise (P = 0.000) postoperatively, however, similarly in both the groups (P = 0.993). Amylase remained unchanged during CPB (P = 0.625) and rose postoperatively (P = 0.000), without any intergroup differences (P = 0.667). The C-reactive protein increased only marginally during CPB to be followed by a considerable elevation (P = 0.000) during the ICU stay, especially in the static group (P = 0.049) (Fig. 2 ).
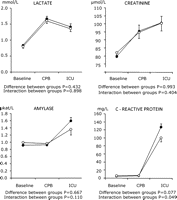
Influence of static or dynamic blood-flow control on the lactate, creatinine, amylase and the C-reactive protein level measured at baseline, during CPB and in the intensive care unit (ICU). Statistical results refer to analysis of variance (ANOVA), repeated measures. (-○-) Dynamic group; (-●-) static group.
A few patients received epinephrine (n = 6 vs 4) (P = 0.741) and/or norepinephrine (n = 5 vs 7) (P = 0.549), whereas the great majority was medicated with phenylephrine (2.48 ± 0.24 vs 2.35 ± 0.17 mg) (P = 0.662) in the dynamic and static groups, respectively. The net water balance during CPB (volume of clear fluids − urine output) was somewhat lower in the dynamic group compared to the static group: 1977 ± 48 versus 2153 ± 88 ml (P = 0.080). No patient received blood transfusion during CPB.
Outcome with respect to postoperative blood loss and ventilator time, including stay in ICU and hospital, was without any statistically significant group differences. All patients survived.
4 Discussion
Use of static blood-flow control during CPB markedly reduced oxygen delivery and thereby the amount of oxygen readily available at the tissue level for consumption. The oxygen balance was however still preserved by compensatory increase of the oxygen extraction rate manifested as a significantly lower SvO2 level at all points. The threshold at which SvO2 no longer can meet oxygen demand is not defined [10]. However, venous oxygen extraction rates exceeding 50% are described to increase the risk for tissue hypoxia and organ dysfunction [9], which in turn may have serious implications upon clinical outcome [11]. At normal physiological conditions a more modest extraction rate is anticipated. The reference target for SvO2 is usually set to 75% [9], which entails larger margins for safety and safeguard of organ function.
The two most vital components in the control of oxygen delivery during CPB are pump rate and the haemoglobin concentration [12]. If pump rate is being kept constant, that is, based on the patient’s BSA [3], the oxygen delivery will more or less independently vary with changes in the haemoglobin concentration. The use of extracorporeal circulation and strategies to compensate for surgical bleeding will both enforce various degrees of haemodilution, with subsequent reductions in haemoglobin availability and oxygen-carrying capacity [13]. Several reports have proposed anaemia encountered during CPB to represent a significant and independent predictor for adverse outcomes [14], ranging from specific [13,15] to more generalised organ deficits [16]. It remains however unclear if these complications are exclusively linked to anaemia or should be attributed to relative hypoxia or deficiency in oxygen delivery or other co-morbidities difficult to control for. Animal experiments indicate that extreme haemodilution using haematocrit levels down to 12% are feasible and compatible with intact systemic and cerebral circulation [5,8]. The safe level of haemodilution in the clinical setting is still debatable and refers to a number of reports, mostly retrospective in design, why general guidelines are difficult to formulate [17]. Since anaemia also reduces oxygen-carrying capacity, the discussion should perhaps more focus on limitations in oxygen delivery, rather than effects of haemodilution and anaemia per se[18].
The concept of dynamic blood-flow control guided by the actual SvO2 value will maintain the adequacy of oxygen supply irrespective of variations in the haemoglobin concentration and overall oxygen demand. Despite these apparently theoretical advantages associated with the dynamic flow-control principle; we were unable to demonstrate any clear benefits with respect to organ function or clinical outcome in our series of patients. The most likely reason is patient selection. The great majority was low-risk patients. Results may have turned out differently in a cohort exposed to prolonged and more complicated surgery. High-risk patients are always more vulnerable to the exposure of CPB and where the consequences of static blood-flow control may have a significant negative impact. Ranucci et al. has shown [15] how inadequacy in oxygen delivery may critically interfere with the normal function of the kidneys. The authors proposed low pump rate as the key component for the deficient oxygen supply.
The significantly lower systemic vascular resistance identified in the dynamic group cannot be explained by inhomogeneous variations in mean arterial pressure, oxygen demand or vasoactive drugs usage between groups. Mechanisms, which as a rule interfere with the control of systemic vascular tone, include myogenic, shear-dependent, metabolic and neural responses [19]. To what extent these components contributed to the observed lower SVR in the dynamic group remains speculative. The metabolic component seems less likely, since the oxygen demand appeared equal between the groups. A combined myogenic or shear-dependent interference seem more plausible. Furthermore, a number of powerful vasoactive substances released into the circulation during CPB also play a paramount role, where the concentration of nitric oxide has been described to correlate with changes in SVR [20]. It should also be emphasised that peripheral circulation and vascular tone in the normal case is intimately linked to variations in cardiac output. However, in the odd situation of CPB, physiological control of cardiac output is set aside and replaced by a blood pump. Rapid increases of pump rate may therefore physically distend peripheral vasculature and enforce a condition of lower vascular resistance superimposed on normal regulatory mechanisms [19]. In agreement with our observations it has been shown that SVR is decreasing in an exponential fashion with increasing pump rate [21]. If this is a normal reaction to variations in pump rate is disputed. With inclusion of the critical closing pressure in the mathematical calculation of SVR, it seems like systemic vascular resistance is independent of variations in pump rate or blood flow [21]. The delicate matter of flow versus resistance behaviour during CPB needs to be looked into more thoroughly.
The clinical significance of SvO2 monitoring per CPB has so far not been proven. Experiences from the intensive care situation imply that monitoring of SvO2 serves as an important marker of critical illness and assists the clinician to optimise therapeutic interventions. Inclusion of the SvO2 information has been shown to decrease the mortality in a group of patients in septic shock [9]. These findings indicate that online monitoring of SvO2 per CPB could be associated with the same premises for improvements in patient outcome.
Gollan [22] suggested already some 50 years ago the use of SvO2 as guidance for systemic blood-flow requirements during CPB. At that time measurement of SvO2 was based on intermittent blood samples, which from practical reasons restricted clinical use. Today, when SvO2 is monitored online, the practical hindrance is no longer an issue. Nonetheless, SvO2 has failed acceptance as reference for blood-flow control. The reason may seem obscure. Some criticise SvO2 of being misleading, since it only reflects the mean mixed venous oxygen saturation, with poor correlation with regional oxygenation processes [23,24]. Indeed, SvO2 is not a monitor of regional oxygenation. Oxygen extraction rates vary extensively in-between different organ beds controlled by local regulatory mechanism and priority of blood supply [19]. Using the SvO2 reading to target these variations would seem irrelevant. SvO2 is exclusively a measure of global oxygenation [25]. To modulate the pump rate according to variations in SvO2 will simply adapt the amount of oxygen being delivered to the actual amount of oxygen being needed at the tissue level. The principle of dynamically controlling pump rate has the obvious advantage of being insensitive to factors, which otherwise may critically disturb the delicate balance between oxygen delivery and demand. One factor of tremendous importance is the use of hypothermia. Already at 30 °C, the metabolism is roughly reduced by half, allowing for a pump rate reduction to a similar extent. The influence of body temperature may easily vary the oxygen demand by more than 100% during phases of cooling or re-warming the patient. Use of a static pump rate regimen, with the patient’s body surface as the only reference would under such conditions, probably both over- and under-estimate the actual pump rate or blood-flow requirement considerably.
4.1 Limitations
Patient selection was limited to low-risk coronary bypass surgical patients exposed to relatively short periods of CPB. Episodes of mismatch in oxygen balance were scant in absence of patients with anaemia and episodes of increasing oxygen demand. High-risk patients, emergencies and low-weight patients may have been warranted.
4.2 Conclusions
SvO2-guided dynamic pump flow control per CPB preserves oxygen delivery irrespective of changes in oxygen demand and oxygen-carrying capacity. No effects could be demonstrated as regards organ function or clinical outcome. Further studies are warranted to verify possible organ-protective qualities and effects on outcome.
The present study was funded by the Heart Foundation of Northern Sweden.
References
- oxygen
- coronary artery bypass surgery
- cardiopulmonary bypass
- vascular flow
- hypothermia, natural
- creatinine
- hemoglobin
- amylases
- body surface area
- lactates
- oxygen consumption
- randomization
- safety
- surgical procedures, operative
- weaning
- c-reactive protein
- oxygen delivery
- oxygen extraction
- unit of flow rate
- venous oxygen saturation
- fluid flow