-
PDF
- Split View
-
Views
-
Cite
Cite
Caroline Van De Wauwer, Arne P. Neyrinck, Nele Geudens, Filip R. Rega, Geert M. Verleden, Toni E. Lerut, Dirk E.M. Van Raemdonck, The mode of death in the non-heart-beating donor has an impact on lung graft quality, , European Journal of Cardio-Thoracic Surgery, Volume 36, Issue 5, November 2009, Pages 919–926, https://doi.org/10.1016/j.ejcts.2009.05.010
- Share Icon Share
Abstract
Objective: We hypothesised that the agonal phase prior to cardiac death may negatively influence the quality of the pulmonary graft recovered from non-heart-beating donors (NHBDs). Different modes of death were compared in an experimental model. Methods: Non-heparinised pigs were divided into three groups (n = 6 per group). Animals in group I [FIB] were sacrificed by ventricular fibrillation resulting in immediate circulatory arrest. In group II [EXS], animals were exsanguinated (45 ± 11 min). In group III [HYP], hypoxic cardiac arrest (13 ± 3 min) was induced by disconnecting the animal from the ventilator. Blood samples were taken pre-mortem in HYP and EXS for measurement of catecholamine levels. After 1 h of in situ warm ischaemia, unflushed lungs were explanted and stored for 3 h (4 °C). Left lung performance was then tested during 60 min in our ex vivo reperfusion model. Total protein concentration in bronchial lavage fluid was measured at the end of reperfusion. Results: Pre-mortem noradrenalin (mcg l−1) concentration (baseline: 0.03 ± 0) increased to a higher level in HYP (50 ± 8) vs EXS (15 ± 3); p = 0.0074. PO2 (mmHg) at 60 min of reperfusion was significantly worse in HYP compared to FIB (445 ± 64 vs 621 ± 25; p ≪ 0.05), but not to EXS (563 ± 51). Pulmonary vascular resistance (dynes s cm−5) was initially higher in EXS (p ≪ 0.001) and HYP (NS) vs FIB (15824 ± 5052 and 8557 ± 4933 vs 1482 ± 61, respectively) but normalised thereafter. Wet-to-dry weight ratio was higher in HYP compared to FIB (5.2 ± 0.3 vs 4.7 ± 0.2, p = 0.041), but not to EXS (4.9 ± 0.2). Total protein (g l−1) concentration was higher, although not significant in HYP and EXS vs FIB (18 ± 6 and 13 ± 4 vs 4.5 ± 1.3, respectively). Conclusion: Pre-mortem agonal phase in the NHBD induces a sympathetic storm leading to capillary leak with pulmonary oedema and reduced oxygenation upon reperfusion. Graft quality appears inferior in NHBD lungs when recovered in controlled (HYP) vs uncontrolled (EXS and FIB) setting.
1 Introduction
Lung transplantation is the mainstay therapy for patients with end-stage pulmonary disease refractory to medical treatment. Donors who are declared dead by neurological criteria provide most of the lungs nowadays. However, only 15–30% of the brain-dead donors have lungs that are deemed transplantable [1]. The use of non-heart-beating donors (NHBDs) may be an alternative solution for the persistent problem of organ shortage [2]. Recently, successful transplantation has been reported by several groups worldwide in several case reports or small clinical series from both uncontrolled [3,4] and controlled [5–8] NHBD. NHBD can be classified into four categories according to the Maastricht classification [9]. In category I (dead on arrival) and category II (failed resuscitation), cardiac death occurs unexpectedly outside the hospital and the situation for organ recovery is therefore ‘uncontrolled’. In category III (withdrawal of life support awaiting cardiac arrest) and category IV (cardiac arrest in brain-dead donor), circulatory arrest is anticipated and organs can be recovered under ‘controlled’ circumstances. Exsanguination and myocardial infarction or fibrillation are common causes of death in the uncontrolled NHBD. This may lead to a period of haemodynamic instability prior to circulatory arrest and cardiac death. On the other hand, in patients with irreversible brain damage not fulfilling the brain death criteria where the ventilatory support is withdrawn (controlled NHBD), hypoxia will also result in haemodynamic instability and circulatory stop. Little is known about the impact of pre-mortem instability, the so-called agonal phase, on the quality of the graft prior to retrieval and on its performance after transplantation. Previous experiments have shown that a period of hypotension, followed by circulatory arrest, impairs lung viability [10] and that pre-arrest hypoxic perfusion is less detrimental for the pulmonary allograft than for the cardiac allograft [11]. However, no study so far has compared different modes of cardiac death.
The purpose of this experimental porcine study was to investigate pre-mortem haemodynamic disturbances during the agonal phase and to compare their influence on graft performance after preservation using an isolated lung reperfusion model between animals succumbing from different modes of death (hypoxia vs hypovolaemic shock vs cardiogenic shock).
2 Material and methods
2.1 Experimental groups
Eighteen domestic pigs (n = 6 per group; weight: 37.2 ± 1.1 kg) were randomly divided into three groups. Pigs in the first group were sacrificed by inducing ventricular fibrillation [FIB] with a subxyphoidal needle puncture using a square-pulse generator (amplitude range +15 to −15 V, current ≪300 mA, frequency 50 Hz), resulting in immediate cardiac arrest. After cardiac arrest, the endotracheal tube was disconnected from the ventilator and left open. In the second group, the animals were exsanguinated [EXS] via a catheter in the external jugular vein. Finally, in the third group, hypoxic arrest [HYP] was induced by disconnecting the animal from the ventilator. After 1 h of warm ischaemia, the heart–lung block was explanted and stored on ice for 3 h (4 °C).
2.2 Animal preparation
Animals were pre-medicated with an intramuscular injection of Xylazine (5 ml Xyl-M® 2%, V.M.D. nv/sa, Arendonk, Belgium) and Zolazepam/Tiletamine (3 ml Zoletil® 100, Virbac s.a., Carros, France). The animals were placed in a supine position and intubated with an endotracheal tube 7.5 (Portex Tracheal Tube, SIMS Portex, Ltd. Hythe, Kent, UK) and ventilated with a volume-controlled ventilator (Titus®, Dräger, Lübeck, Germany) with an inspiratory oxygen fraction (FiO2) of 0.5 and a tidal volume of 10 ml kg−1 body weight. Respiratory rate was adjusted to achieve an end-tidal CO2 of 40 mmHg. Positive end-expiratory pressure was set to 5 cm H2O. Anaesthesia was maintained with isoflurane 0.8–1% (Isoba® Vet, Schering – Plough Animal Health, Harefield, Uxbridge, UK) and muscle relaxation with intermittent boli of pancuronium bromide (Pavulon 2 mg ml−1, Organon, Teknika, Boxtel, the Netherlands). A 14-G catheter (Secalon® T, Becton Dickinson Ltd., Singapore) was placed in the right common carotid artery for measurement of the systemic arterial pressure (SAP) and sampling of arterial blood. A 7.5 F Swan-Ganz thermodilution catheter (Baxter Healthcare Corp., Irvine, CA, USA) was inserted through the right external jugular vein into the pulmonary artery. With this catheter, haemodynamic parameters, including pulmonary artery pressure (PAP) and pulmonary capillary wedge pressure (PCWP), were monitored. Haemodynamic parameters (SAP, PAP and PCWP) and aerodynamic parameters (plateau airway pressure and compliance) were continuously monitored and stored on a computer.
After a stabilisation period, the pigs were sacrificed according to the study protocol described above. No heparin was administered in any group. After cardiac arrest in [EXS] and [FIB], the endotracheal tube was disconnected from the ventilator and left open to the air. At the end of the agonal phase, blood samples were taken in [EXS] and [HYP] to analyse catecholamine levels. All cadavers were left untouched for 1 h at room temperature. Temperature of the lung was measured via a probe in the endotracheal tube and rectal temperature was monitored.
All animals received humane care in compliance with the Principles of Laboratory Animal Care, formulated by the National Society for Medical Research and the Guide for the Care and Use of Laboratory Animals, prepared by the Institute of Laboratory Animal Resources, National Research Council, and published by the National Academy Press, revised 1996 (NIH Publication No. 85-23, Revised 1996). The study was approved by the institutional review board on animal research at the Katholieke Universiteit Leuven.
2.3 Preparation of the heart–lung block
After 1 h of warm ischaemia, a sternotomy was performed. The thymic tissue was excised and the pericardium and pleural cavities were widely opened. The lungs were inspected. The pulmonary artery, ascending aorta and caval veins were encircled. Gross thrombi in the pulmonary artery and left atrium were removed as much as possible and the lungs were explanted without flush. After excision of the heart–lung block, the lungs were collapsed and immersed in cold (4 °C) Perfadex® and stored on ice for 3 h.
The lungs in all three groups were prepared in the same way for ex vivo evaluation in the isolated reperfusion system after cold storage. The right lung was separated from the heart–lung block and used as a control. The pulmonary artery was cannulated through the right ventricular outflow tract using a 36-Fr cannula and isolated with a ligature around the catheter distal to the pulmonary valve. A small catheter was placed in the pulmonary artery for measurement of PAP. The ascending aorta was clamped. The left atrium was cannulated through the apex of the left ventricle with a second 36-Fr cannula and secured with a purse-string. Finally, an endotracheal tube nr. 8 was placed in the trachea for ventilation of the pulmonary graft.
2.4 Preparation of the perfusate
Autologous blood (1200 ml) was withdrawn from each animal in [HYP] and [FIB] after circulatory arrest via the catheter in the right external jugular vein and collected in a sterile bag containing 5000 IU of heparin (Natrium Heparin B. Braun, 25 000 IU per 5 ml, B. Braun Medical SA, Jaén, Spain). In [EXS], animals were sacrificed by exsanguination and this blood was collected for the preparation of the perfusate. This whole blood was centrifuged with a Cell Saver (Sequestra 1000, Medtronic Inc., Parker, CO, USA) and washed with saline for 12 min at 5600 rpm. Leucocytes were sequestered using a leucocyte filter (Imugard III-RC, Terumo Europe N.V., Haasrode, Belgium). The remaining red blood cells (350 ml) were then diluted to a haematocrit of 15% with a low potassium dextran solution (Perfadex®, Vitrolife, Göteborg, Sweden) and human albumin (final concentration: 8%, CAF-DCF, Brussels, Belgium). The perfusate was finalised by adding CaCl2 (2.4 ml l−1, 100 mg ml−1), heparin (10 000 IU l−1) and sodium bicarbonate (45 ml l−1, 16.8 g per 250 ml Baxter, Lessines, Belgium). The total volume of the perfusate was 1400 ml.
2.5 Isolated reperfusion circuit
The ex vivo reperfusion system consisted of a hard-shell reservoir (Minimax® Hardshell reservoir, Medtronic, Minneapolis, MN, USA), a centrifugal pump (Bio-medicus, Medtronic), a heater/cooler system (Bio-Cal, Heater Cooler Model 370, Medtronic, Minneapolis, MN, USA) and a hollow fibre oxygenator (Capiox®SX, Terumo, MI, USA) with integrated heat-exchanger. The heating element of the gas exchanger was connected to the heater/cooler system. The left lung and the heart were then placed in a specially designed evaluation box and mounted in the reperfusion system. The cannula in the pulmonary artery was connected to the inflow tubing and the outflow tubing was connected to the cannula in the left atrium.
2.6 Technique of controlled reperfusion and ventilation
Reperfusion of the left lung was started with normothermic (37 °C) oxygenated perfusate (O2: 0.4 l min−1) after de-airing of the inflow tubing. PAP was gradually increased to a maximum of 15 mmHg and the left atrial pressure (LAP) on the outflow was kept at 0 mmHg by adjusting the height of the blood reservoir. This resulted in warming up of the lung and a gradual increase in pulmonary artery flow. Ventilation with a FiO2 0.5 was started when the temperature of the outflowing perfusate reached 34 °C and slowly increased to a tidal volume of 140 ml, a frequency of 14 breaths min−1 and positive end-expiratory pressure (PEEP) of 5 cm H2O. At that moment, the perfusate was partially deoxygenated to a of 50–60 mmHg with a gas mixture of CO2 (8%), O2 (6%) and N2 (86%).
2.7 Assessment of the graft
Forty minutes after the onset of reperfusion, the temperature of the lung parenchyma reached 37.5 °C. At this moment, functional graft parameters were recorded up to 1 h. PAP (mmHg) was measured via an 18-G catheter inserted in the main pulmonary artery. The LAP (mmHg) was measured on the outflow line. An electromagnetic flow probe (FF 100T 10-mm probe, Nihon Kohden, Tokyo, Japan) was inserted in the tubing on the inflow line for continuous measurement of the pulmonary artery flow (PAF; l min−1). Pulmonary vascular resistance (PVR) was calculated using the formula: PVR = [PAP − LAP] × 80/PAF and expressed in dynes s cm−5. Dynamic lung compliance (Compl) (ml cm−1 H2O) and plateau airway pressure (Plat AwP) (cm H2O) were recorded. PO2 and PCO2 were continuously measured in the perfusate via probes (Terumo CDI™, 500 shunt sensor, Leuven, Belgium) on the outflow tubing using an inline blood gas analyser (CDI™ 500, Terumo, Borken, Germany). Oxygenation capacity was calculated using the PO2/FiO2 ratio (mmHg).
Temperature (°C) of the inflowing and outflowing perfusate was continuously measured, the last being considered as the graft temperature. All data were recorded online and stored on a central server (Datex AS/3 and S5 collect 3.0 Software, respectively, Datex-Ohmedia, Helsinki, Finland).
At the end of the reperfusion, both right and left lung were dried in an oven at 80 °C for 48 h to a constant weight and their wet-to-dry (W/D) ratio was calculated and used as a parameter of pulmonary oedema.
2.8 Biochemical analysis of plasma catecholamines
The catecholamines were measured in plasma, using high-performance liquid chromatography with electrochemical detection (HPLC-ED). Activated alumina was used to extract the catecholamines from plasma. After a wash step, the analytes were eluted with an acetic acid solution and separated on a reversed-phase column in ion-pair chromatography mode. Concentrations were determined using calibration curves covering a range from 0 to 2000 ng l−1. The lower limit of the quantification was 0.05 ng l−1.
2.9 Biochemical analysis of total protein in bronchial lavage fluid
Bronchial lavage (BL) was performed in the right lung after explantation, and in the left lung immediately at the end of the reperfusion. Sterile saline measuring 25 ml, at room temperature, was instilled in the bronchus and aspirated with gentle suction after 1 min in a standardised way. The returned fraction was centrifuged at 3500 rpm for 10 min at 4 °C. The supernatant was collected for further analysis and stored at −80 °C.
Total protein was measured in the BL supernatant by means of the biuret method (Roche Diagnostics GmBH, Mannheim, Germany). Protein reacts in an alkaline solution with divalent copper to form a purple-coloured biuret complex. The colour intensity is measured photometrically and is proportional to the protein concentration. The lower detection limit was 2 g l−1 with an upper limit of 150 g l−1.
3 Statistical analysis
Data were analysed using Graphpad Prism 4 (San Diego, CA, USA). Graft parameters between study groups were compared using a one-way analysis of variance with a Newman–Keuls multiple comparison test. A paired t-test was used to test for significant difference for W/D between the left perfused and the right non-perfused lung. An unpaired t-test was used to test for significant difference in catecholamine levels. A p-value ≪0.05 was considered as significant. Data regarding pulmonary graft function and catecholamines are expressed as mean ± standard error of the mean (SEM). All other data are expressed as mean ± standard deviation (SD)
4 Results
4.1 Study groups
Baseline parameters prior to sacrifice of the animals in these groups are depicted in Table 1 . There was no significant difference for animal weight, PAP, Compl and .

Baseline parameters prior to circulatory arrest in the three animal groups.
All animals in FIB were pulseless immediately after needle puncture as a result of myocardial fibrillation. Animals undergoing exsanguination (EXS), were pulseless after 45 ± 11 min. The agonal phase was characterised by changes in heart rate and in blood pressure. Initially, there was a simultaneous decrease in heart rate and blood pressure (hypotensive bradycardia). In the next stage, the heart rate increased but the blood pressure remained the same (hypotensive tachycardia). This was followed by a drop in the heart rate and a further decrease in blood pressure until pulselessness.
In the hypoxic arrest group (HYP), animals were pulseless after 13 ± 3 min. After disconnection of the endotracheal tube, there was an increase in the heart rate and in blood pressure (hypertensive tachycardia). Thereafter, both heart rate and blood pressure dropped continuously until loss of electrical activity. The haemodynamic changes during the agonal phase in one animal for EXS and HYP are demonstrated in Fig. 1A and B , respectively.
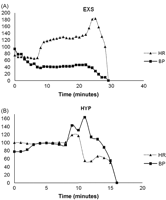
Example of haemodynamic changes in heart rate (HR) and in arterial blood pressure (BP) during the agonal phase in 1 animal from each study group. (A) Exsanguination. (B) Hypoxic cardiac arrest.
Pulmonary graft characteristics before reperfusion in the three groups are compared in Table 2 . There was no significant difference between the three groups for the warm ischaemic interval, cold ischaemic interval and the temperature of the graft at the end of the cold ischaemic period. The time necessary to warm up the lung to 34 °C at the time of reperfusion was longer in EXS and HYP compared to FIB (p = 0.017).

Pulmonary graft characteristics before reperfusion in the three study groups.
4.2 Catecholamine concentrations
Noradrenaline levels (mcg l−1) taken immediately before cardiac arrest were significantly higher in HYP (50 ± 8) vs EXS (15 ± 3) (p = 0.0074) (Fig. 2A ). The pre-mortem adrenaline levels were also higher in HYP compared to EXS, although the difference did not reach significance (45 ± 15 and 14 ± 4, respectively) (Fig. 2B). Noradrenaline and adrenaline levels in HYP and EXS were significantly higher pre-mortem compared to the baseline values (0.03 ± 0) (p ≪ 0.05) (Figs. 2A and B).
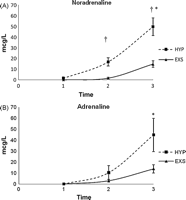
Catecholamine concentrations during the agonal phase. (A) Noradrenaline: †p ≪ 0.05 HYP vs EXS, *p ≪ 0.05 pre-mortem vs baseline in HYP and EXS. (B) Adrenaline: NS between groups, *p ≪ 0.05 pre-mortem vs baseline in HYP and EXS.
4.3 Pulmonary graft function
4.3.1 Pulmonary vascular resistance
PVR (dynes s cm−5) was higher in EXS (p ≪ 0.001) and HYP (NS) compared to FIB at 40 min of reperfusion (15824 ± 5052 and 8557 ± 4933 vs 1482 ± 61). This difference disappeared during longer reperfusion (1531 ± 174 and 1369 ± 181 vs 1435 ± 95, respectively, at 60 min) (p > 0.05) (Fig. 3A ).
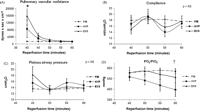
Pulmonary graft function (mean ± SEM) from 40 min until 60 min after start of ex-vivo reperfusion. FIB: ventricular fibrillation, EXS: exsanguination and HYP: hypoxic cardiac arrest. (A) Pulmonary vascular resistance: *p ≪ 0.001 EXS vs FIB at 40 min. (B) Dynamic lung compliance: NS between groups. (C) Plateau airway pressure: NS between groups. (D) Oxygenation capacity (PO2/FiO2): †p ≪ 0.05 HYP vs FIB at 60 min.
4.3.2 Dynamic lung compliance
There was no difference in Compl (ml cm−1 H2O) between FIB, EXS and HYP during the reperfusion (Fig. 3B), but this parameter appeared more stable in FIB.
4.3.3 Plateau airway pressure
Plat AwP (cm H2O) was initially higher in EXS and HYP compared to FIB. Although the difference did not reach significance (18 ± 3 and 16 ± 2 vs 13 ± 1, respectively), this normalised later on (Fig. 3C).
4.3.4 Oxygenation capacity
PO2 (mmHg) at 60 min of reperfusion was significantly worse in HYP vs FIB (445 ± 64 vs 621 ± 25; p ≪ 0.05) but not when compared to EXS (563 ± 51). There was also no significant difference between FIB and EXS (Fig. 3D).
4.4 Protein concentrations
The total protein concentration (g l−1) in the BL fluid of the left lung was higher, although not significant in HYP and EXS compared to FIB (18 ± 6 and 13 ± 4 vs 4 ± 1, respectively).
4.5 W/D ratio
The W/D ratio of the perfused left lung was significantly higher in HYP compared to FIB (5.2 ± 0.3 vs 4.7 ± 0.2, p = 0.041) but not to EXS (4.9 ± 0.2). There was no significant difference in W/D ratio between the three groups for the non-perfused right lung. W/D ratio was significantly lower in FIB and in EXS in the left lung compared to the right lung. There was no difference for HYP (Table 3 ).
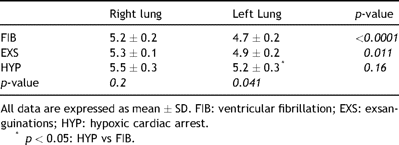
W/D ratio in the right (non-perfused) and left (perfused) lung in all study groups.
5 Discussion
The goal of this study was to investigate the impact of pre-mortem instability and to compare different modes of death in the NHBD on graft performance. We therefore compared animals succumbing to cardiac arrest resulting from ventricular fibrillation, exsanguination or hypoxia. We demonstrated that lungs recovered from hypoxic animals were of inferior quality with significantly worse oxygenation at 60 min of reperfusion compared to lungs retrieved from animals with sudden death by myocardial fibrillation without agonal period. W/D ratio was also higher in HYP compared to FIB. PVR was also higher in HYP (NS) and EXS (p ≪ 0.001) compared to FIB. As a result, the time necessary to warm the lung up in the ex vivo circuit was significantly longer in HYP and EXS vs FIB. Total protein concentration was higher, although not significant in HYP and EXS vs FIB. Pre-mortem noradrenaline concentration was significantly higher in HYP compared to EXS. These findings suggest that the pre-mortem agonal phase during hypoxia induces a catecholamine storm, leading to capillary leak with pulmonary oedema and reduced oxygenation upon reperfusion much worse than during hypovolaemia.
Cerebral herniation in the heart-beating donor is initially characterised by a hyperdynamic state with hypertension and tachycardia associated with changes in the circulating plasma catecholamines. This is followed by neurogenic hypotension. There is also evidence that systemic inflammatory pathways are activated during this process. The lung is susceptible to these changes resulting in neurogenic pulmonary oedema and in an acute inflammatory lung injury [12,13].
The use of lungs recovered from NHBD has recently been propagated as an alternative to overcome the critical organ shortage [14]. There are, however, still several concerns to the use of the lungs from NHBD [2]. The influence of the pre-mortem instability is one of them. It is hypothesized that the injury to the graft in the pre-mortem agonal period could be more noxious than the injury that occurs during the warm ischaemic interval prior to cold preservation. The effect of pre-mortem instability in the NHBD on the outcome has previously been addressed by some research groups [10,11]. However, no study compared the different modes of cardiac death. When our study was designed, we decided to compare the different situations that occur in patients dying from cardiac death qualifying as potential NHBD. We therefore looked at scenarios of sudden death following myocardial fibrillation as well as longer agonal periods prior to death resulting from hypoxia or hypovolaemia. These scenarios reflect what can happen in the clinical situation for both uncontrolled [FIB and EXS] and controlled [HYP] donors after cardiac death.
Secher and colleagues described haemodynamic events during reversible hypovolaemic shock [15]. In the first stage, an increase in heart rate is associated with a normal or slightly increased blood pressure. This is followed by a decrease in heart rate and blood pressure. In the third stage, blood pressure falls further and tachycardia is present. This stage can proceed to irreversible shock with cardiac arrest. We observed similar haemodynamic changes during the agonal phase in our animals that were exsanguinated.
After disconnection of the endotracheal tube in HYP, animals developed a marked increase in heart rate and blood pressure. This was paralleled with a significant increase in noradrenaline. Thereafter, both heart rate and blood pressure dropped until loss of electrical activity. This was also reported in a study by DeBehnke and colleagues where a canine model of asphyxial arrest with pulseless electrical activity was used [16]. Animals went through a characteristic pattern of tachycardia and hypertension, followed by bradycardia and hypotension. Erasmus and colleagues also reported a period of central hypertension preceding cardiac arrest in NHBD pigs sacrificed after ventilator switch-off [17].
In an isolated rat reperfusion model, Tremblay and co-workers investigated the influence of haemodynamic instability (mean blood pressure 30–40 mmHg) during 1 h before death in combination with different lengths of warm ischaemia (0, 2 or 3 h), followed by cold flush preservation [10]. Haemorrhagic oedema developed during reperfusion in lungs recovered from animals after 1 h of haemorrhagic shock followed by 2–3 h of warm ischaemia. Lungs that were not subjected to an additional period of warm ischaemia after circulatory arrest did better. This study suggests that the combination of 1 h hypotension and 2 h of warm ischaemia is deleterious to the lung. Our group has previously demonstrated that the warm ischaemia tolerance in the deflated lung is limited to 60–90 min [18,19].
In the present study, exsanguination was followed by 1 h of warm ischaemia. The lungs were harvested without flush and stored on ice for 3 h. The left lung was than evaluated in an ex vivo reperfusion system. Gas exchange was slightly worse in EXS when compared to FIB but there was no significant difference. There was also no significant difference for compliance, PVR and Plat AwP.
In a rabbit model of isolated lung perfusion, Mauney et al. found that pulmonary allografts after hypoxic arrest and 20 min of warm ischaemia showed no significant differences in PVR, oxygenation, airway resistance and baseline PAP after 45 min of reperfusion [11]. Animals were heparinised before hypoxic arrest and lungs were not exposed to cold ischaemia. In the present study, no heparin was administered before hypoxic arrest and lungs were exposed to cold storage. Oxygenation was significantly worse after 60 min of reperfusion in HYP compared to FIB. The Groningen group also reported impaired lung function in an ex vivo pig lung perfusion study where animals were sacrificed by ventilator switch-off, reflecting the clinical setting of controlled NHBD. This was followed by 1 h of warm ischaemia and topical cooling. Lungs were preserved for 6 h using ex vivo lung perfusion. The authors hypothesized that the hypertensive period before cardiac arrest causes endothelial damage by mechanical stretching with release of pro-inflammatory cytokines [17].
We observed a higher PVR in HYP and EXS compared to FIB at the start of the reperfusion. Hypoxic pulmonary vasoconstriction as in HYP is a response to a decreased alveolar oxygen tension, which results in vasoconstriction of the small muscular pulmonary arteries increasing the PVR. Haemorrhagic shock as in EXS is characterised by hypovolaemia and hypoxia resulting in pulmonary vasoconstriction. We hypothesize that the longer duration of the agonal phase in EXS compared to HYP resulted in an initial higher PVR and that the increase of the catecholamines has less influence on PVR in the lungs. Reperfusion was performed in a controlled setting with a maximum inflow pressure of 15 mmHg. The high PVR in HYP and EXS resulted in a reduced flow through the lung and therefore necessitating a longer time to warm up the lung.
Elevated catecholamines and, mainly norephinephrine, cause an increase in total peripheral vascular resistance and in right ventricular systolic pressure leading to pulmonary congestion. It can also increase pulmonary venous tone with increased hydrostatic pressure in the pulmonary capillaries resulting in pulmonary oedema. Norephinephrine stimulates pro-inflammatory cytokines resulting in increased permeability of the alveolar–capillary barrier and pulmonary oedema. This was demonstrated experimentally in a study where rats received a continuous intravenous infusion of norephinephrine [20] and in clinical studies after lung transplantation [21]. Similar changes are described to explain pulmonary events in neurogenic pulmonary oedema after brain death [22–24] and the inflammatory changes in patients with acute lung injury [25]. We also found a significantly higher level of catecholamines in the period preceding death in HYP compared to EXS. The W/D ratio of the perfused left lung and protein levels were also higher in HYP. This suggests that the increase of catecholamines during the agonal phase after hypoxic cardiac arrest may have an important impact on graft performance.
As we were interested in the sole effect of the agonal phase on graft performance, no drugs were administered during the agonal phase or after circulatory arrest and the lungs were retrieved without pulmonary flush.
The present study suffers from several limitations to translate our conclusions to the clinical situation in NHBD. Firstly, the reperfusion model and therefore the period to evaluate graft performance are limited in time. The present findings, therefore, need to be confirmed in a transplant model. Secondly, animals with healthy lungs were used in this study. It is most likely that this is completely different in the clinical situation. Controlled NHBD dying from hypoxic cardiac arrest after ventilatory switch-off may already have suffered some form of lung injury from barotrauma, aspiration or infection when ventilated in the period after severe insult to the brain. Uncontrolled donors dying from hypovolaemic shock may also develop lung injury as a result of direct trauma or resuscitation manoeuvres with cardiac massage, administration of vasopressors and fluid resuscitation. Most patients dying from cardiogenic shock succumb to myocardial infarction. These patients do not always develop sudden cardiac arrest from myocardial fibrillation as was the case in the present study. Therefore, the agonal period and thus inflammatory lung injury in the clinical situation may also contribute to the outcome.
In conclusion, in this experimental pig lung reperfusion study, we have demonstrated that the pre-mortem agonal phase after switch-off procedure induces a catecholamine storm leading to capillary leak with pulmonary oedema and reduced oxygenation upon reperfusion. Pulmonary graft quality appears to be inferior when recovered from controlled (HYP) vs uncontrolled NHBD (EXS and FIB). Therefore, long periods of haemodynamic instability after ventilator switch-off should raise concerns in a clinical setting. Further studies are needed to identify an acceptable period whereby lungs can be safely transplanted in the setting of controlled donation.
Presented at the 15th European Conference on General Thoracic Surgery, Leuven, Belgium, June 3–6, 2007.
This work is supported by grants from the Katholieke Universiteit Leuven (OT/03/55) and Fund for Scientific Research – Flanders (FWO G.0576.06). Dirk Van Raemdonck is a senior research fellow of the Fund for Scientific Research – Flanders (FWO G.3C04.99).