-
PDF
- Split View
-
Views
-
Cite
Cite
Davide Ricci, Ari A. Mennander, Linh D. Pham, Vinay P. Rao, Naoto Miyagi, Guerard W. Byrne, Stephen J. Russell, Christopher G.A. McGregor, Non-invasive radioiodine imaging for accurate quantitation of NIS reporter gene expression in transplanted hearts, European Journal of Cardio-Thoracic Surgery, Volume 33, Issue 1, January 2008, Pages 32–39, https://doi.org/10.1016/j.ejcts.2007.09.033
- Share Icon Share
Abstract
Objective: We studied the concordance of transgene expression in the transplanted heart using bicistronic adenoviral vector coding for a transgene of interest (human carcinoembryonic antigen: hCEA – beta human chorionic gonadotropin: βhCG) and for a marker imaging transgene (human sodium iodide symporter: hNIS). Methods: Inbred Lewis rats were used for syngeneic heterotopic cardiac transplantation. Donor rat hearts were perfused ex vivo for 30 min prior to transplantation with University of Wisconsin (UW) solution (n = 3), with 109 pfu/ml of adenovirus expressing hNIS (Ad-NIS; n = 6), hNIS-hCEA (Ad-NIS-CEA; n = 6) and hNIS-βhCG (Ad-NIS-CG; n = 6). On postoperative day (POD) 5, 10, 15 all animals underwent micro-single photon emission computed tomography/computed tomography (SPECT/CT) imaging of the donor hearts after tail vein injection of 1000 μCi 123I and blood sample collection for hCEA and βhCG quantification. Results: Significantly higher image intensity was noted in the hearts perfused with Ad-NIS (1.1 ± 0.2; 0.9 ± 0.07), Ad-NIS-CEA (1.2 ± 0.3; 0.9 ± 0.1) and Ad-NIS-CG (1.1 ± 0.1; 0.9 ± 0.1) compared to UW group (0.44 ± 0.03; 0.47 ± 0.06) on POD 5 and 10 (p < 0.05). Serum levels of hCEA and βhCG increased in animals showing high cardiac 123I uptake, but not in those with lower uptake. Above this threshold, image intensities correlated well with serum levels of hCEA and βhCG (R2 = 0.99 and R2 = 0.96, respectively). Conclusions: These data demonstrate that hNIS is an excellent reporter gene for the transplanted heart. The expression level of hNIS can be accurately and non-invasively monitored by serial radioisotopic SPECT imaging. High concordance has been demonstrated between imaging and soluble marker peptides at the maximum transgene expression on POD 5.
1 Introduction
Advances in nuclear imaging techniques have made molecular imaging of gene transduction feasible with the use of reporter genes like herpes simplex virus thymidine kinase (HSVtk), human norepinephrine transporter (hNET) and human sodium/iodide symporter (hNIS). Ideally, the reporter gene is expressed on human cells but not on the organ being targeted and is therefore non-immunogenic.
Sodium iodide symporter (NIS) is an integral plasma membrane glycoprotein that mediates active iodide transport into the thyroid follicular cells, the first step in thyroid hormone biosynthesis. NIS-mediated thyroidal iodide transport from the bloodstream to the colloid is a vectorial process made possible by the selective targeting of NIS to the basolateral membrane. NIS also mediates active iodide transport in other tissues, including salivary glands, gastric mucosa, and lactating mammary glands, in which it translocates iodide into the milk for thyroid hormone biosynthesis by the nursing newborn. NIS provides the basis for the effective diagnostic and therapeutic management of thyroid cancer and its metastases with radioiodide.
Now that NIS has been cloned and characterized, it may be possible to develop novel strategies to differentially modulate NIS expression and/or activity, enhancing it in target tissues and impeding it in others [1,2].
A vital step in transgenic animal studies and gene therapy clinical trials is the ability to monitor the extent of transgene expression. Therefore, it is pertinent to develop techniques non-invasively and repetitively determine the location, duration, and magnitude of transgene expression in living animals. Several studies and clinical trials have already successfully demonstrated the possibility to use therapeutic gene encoded into viral vectors but in none has it been possible to monitor in vivo gene expression [3–5]. In any gene therapy study we should know the number, distribution and fate of the genetically modified cells, and the kinetics of production of the therapeutic protein over time.
It is possible to use NIS as an imaging reporter gene to monitor the expression profile of the transgene in transgenic animal models. The effectiveness of NIS as a non-invasive reporter gene has already been validated by several studies in tumor models of gene transfer [6,7].
The expression of NIS on mammalian thyroid follicular cells makes it an attractive reporter gene due to the potentially attenuated immune response to the gene product. In addition the absence of NIS on the cardiomyocytes makes it an interesting myocardial reporter gene. The transduction of NIS encoded into an adenovirus did not determine myocardial injury or dysfunction in previous in vitro studies [8].
In this study we sought to establish the validity of a novel approach to quantitative monitoring transgene expression in the transplanted heart. The approach is to use a vector coding both for the transgene of interest and for a marker transgene whose expression level can be determined by radioisotopic imaging studies. If there is concordance between the expression levels of the two transgenes, the expression level of one can be inferred from the measured expression level of the other.
The confirmation of this concordance would lead to future applications of cardiac therapeutic genes with the possibility to monitor gene expression over time. In this way we could easily identify the reasons for treatment failure, whether due to inadequate transduction of target cells/inadequate virus dose (little or no gene expression), inadequate virus replication (persistent low level or falling gene expression) or myocardial cell resistance to virus-mediated effect despite adequate virus spread (continuously rising expression).
We used bicistronic adenoviral vectors coding for hNIS and either the soluble extracellular domain of human carcinoembryonic antigen (hCEA) or the beta chain of human chorionic gonadotropin (βhCG). Non-invasive imaging of radioiodine uptake and serum quantification of soluble markers were performed with the use of computed tomography (CT) co-registered with micro-SPECT [9] following adenoviral gene transfer in a rat model of cardiac transplantation using three adenoviral vectors: Ad-hNIS, Ad-hNIS-hCEA and Ad-hNIS-βhCG.
2 Materials and methods
Inbred male Lewis rats (250–350 g, Harlan®, IN) were used as donors and recipients for syngeneic heterotopic heart transplants. Procedures and handling of animals were reviewed and approved by the Institutional Animal Care and Use Committee of the Mayo Clinic and Foundation in compliance with ‘Principles of Laboratory Animal Care’ formulated by the National Society for Medical Research and the ‘Guide for the Care and Use of Laboratory Animals’ prepared by the Institute of Laboratory Animal Resources and published by the National Institutes of Health (National Institutes of Health publication No. 86-23, revised 1996).
2.1 Recombinant adenovirus production
Human CEA, βhCG and hNIS were ligated into human cytomegalovirus (CMV) shuttle vector. Once in the CMV vector, the CMV promoter hCEA/hCG gene was ligated into an E1/E3 deleted adenoviral serotype 5 (Ad) vector at E3 position within Csp451 site. Next, CMV promoter-hNIS was ligated into Ad vector at E1 position after digesting both with I-CeuI and PI-SceI to make Ad-hCEA3-NIS1 and Ad-hCG3-NIS1. All viral vectors were purified using standard methods of ethanol precipitation, phenol–chloroform extraction, mini-prep, and gel extraction. All steps were screened using gel electrophoresis to ensure all reactions were carried out properly and had the desired results.
Once successful cloning and purification of the Ad vector had been completed, the vector was linearized using Pac-1 and transfected into 293 human embryonic cells. The 293 cells were infected using a modified protocol from the Polyfect transfection kit. After well-developed plaques were formed, the cells were harvested and went through three rounds of freeze/thawing before being applied to 293 cells again. This step was done three times in order to amplify the virus. Once this was completed, the virus was rescued using cesium chloride extraction methods. This was followed by using dialysis against 10% glycerol in phosphate buffer saline (PBS) twice in order to collect the purified virus.
The purified virus was diluted in cold University of Wisconsin (UW) solution at a concentration of 109 pfu/ml just prior to ex vivo perfusion of donor hearts. Viral titers were determined by plaque assay and expressed as plaque forming units per ml (pfu/ml).
2.2 Donor operation
The donor hearts were harvested as previously described [10]. Briefly, after anesthesia (pentobarbital sodium 70 mg/kg administered intraperitoneally), a bilateral thoracotomy was performed and 200 U of aqueous heparin was injected into the inferior vena cava. The innominate artery was cannulated with a 24-gauge cannula, and the venae cavae and pulmonary veins were ligated en bloc with 6-0 silk. The aorta was clamped distal to the cannula, and the heart was arrested with an infusion of cold UW into the aortic root through the cannula placed into the innominate artery. After harvesting, the heart was stored in the same cardioplegic solution at 4 °C.
2.3 Experimental groups and gene transfer
Twenty-one transplants were performed within four groups. In the first group (n = 3), the donor hearts harvested as previously described [10] were perfused for 30 min at 4 °C with 5 ml of UW solution using the Langendorff perfusion apparatus. In the second group (n = 6), donor hearts were perfused with 5 ml of Ad-NIS diluted in UW solution at a concentration of 1 × 109 pfu/ml under the same conditions. In groups 3 and 4 (n = 6 in each group) donor hearts were perfused respectively with Ad-NIS-CEA and Ad-NIS-CG following the same protocol. Hearts from all groups were then stored in UW solution at 4 °C while the recipient was prepared. All the animals underwent micro-SPECT/CT imaging of the donor hearts after tail vein injection of 1000 μCi 123I on postoperative day (POD) 5, 10 and 15 following gene transfer. On the same postoperative days 400 μl of blood sample were obtained for hCEA and βhCG serum quantification. A control group using only the adenoviral vector with no gene encoded was not included in this study because in a previous study from our group no differences were found in iodine uptake between hearts perfused with solution containing the adenovirus + no gene (Ad-Null) and only UW perfused hearts.
2.4 Recipient operation
Following anesthesia (pentobarbital sodium 70 mg/kg administered intraperitoneally) a midline laparotomy was performed and the abdominal aorta and the inferior vena cava exposed below the origin of the renal vessels. The donor heart was transplanted into the recipient by end-to-side anastomoses of the aorta and the pulmonary artery to the abdominal aorta and inferior vena cava, respectively, using 8-0 monofilament sutures. During surgery, the heart was wrapped in gauze and kept cold by use of topical ice-cold saline solution. All rats received analgesia postoperatively (Buprenorphine administered subcutaneously) and recovered with oxygen in a warm environment. Function of the graft was checked daily by palpation of the beating transplanted heart.
2.5 Imaging with micro-SPECT
All animals were imaged on POD 5, 10 and 15, under sedation (intraperitoneal pentobarbital 50 mg/kg). 37 MBq of 123I was injected into the tail vein and micro-SPECT/CT imaging commenced after 20 min. Syringe activity was measured prior to and post injection to quantify the injected dose accurately. Micro-SPECT images were obtained under a high-resolution gamma camera (X-SPECT, Gamma Medica-Ideas Inc., CA) with a low energy high-resolution parallel hole collimator with 64 projections at a rate of 10 s per projection and an acquisition time of 13 min 46 s. CT images were obtained under a circular orbit at a thickness of 50 μM per slice under the same scanner without moving the animal in between. This ensured accurate co-registration of the axial CT images with the micro-SPECT maps.
2.6 In Vivo image analysis
SPECT and CT images were stored and pixel-intensity of the images was quantified as described in earlier studies [9]. Briefly, the regions of interest (ROI) were defined manually around the heart shadows on the reconstructed axial tomographic images in all sections in which the heart was visualized, and quantification performed on the co-registered micro-SPECT images. Cumulative pixel counts, within the defined ROI, were converted to activity using a conversion factor of 1.63 × 10−7 MBq/measured count derived from our previous studies. Values were expressed as a percentage of the injected dose per gram of heart tissue (%ID/g).
Measurements were obtained by an independent observer blinded to the origin of the images. The threshold value for image intensity (%ID/g) was estimated from the UW only perfused hearts data pooled across the three time points (POD 5, 10 and 15), which represented the range of baseline amount. Since this cut-off served to differentiate baseline versus virus-induced %ID/g, the maximum value from the baseline data plus one standard deviation was chosen as the threshold value. Serum quantification of soluble markers hCEA and βhCG was performed on blood collected from all the recipients on POD 5, 10 and 15 before each 123I injection and micro-SPECT/CT imaging. Following the last imaging procedure, the rats were euthanized by exsanguination and the transplanted hearts explanted and weighed.
2.7 Anti-hCEA and anti-βhCG antibody screening
Anti-hCEA and anti-βhCG antibodies were analyzed by ELISA. Wells were coated overnight at 4 °C with 200 ng/ml hCEA or βhCG (Scripps Laboratory, San Diego, California). The plates were washed and serial dilutions of rat serum were added. Bound antibodies were detected using horseradish peroxidise-conjugated antibodies and substrate [11]. The test was performed on all the serum samples collected at POD 5, 10 and 15.
2.8 Statistics
We assessed the correlation of %ID/g with hCEA and βhCG both at each time point and overall using univariate linear regression. Specifically, we modeled %ID/g using hCEA as the predictor among Ad-NIS-CEA rats and, separately, βhCG as the predictor among Ad-NIS-CG rats. The model p-value and R2 value were reported. The sensitivity of each parameter, stratified by day, was computed using known cut-off values for hCEA and βhCG serum levels, and an estimated cut-off value for %ID/g. The cut-off value for presence of viral %ID/g was estimated from the UW group (control) data pooled across the three time points, which represented the baseline range. Since the cut-off value would serve to differentiate baseline versus virus-induced %ID/g, the maximum value from the baseline data plus one standard deviation was chosen as the estimate. For those in the three Ad-NIS groups, sensitivity of %ID/g was computed as the percentage of rats over the threshold of 0.6. For hCEA, sensitivity was calculated as the percentage of rats in the Ad-NIS-CEA group above the cut-off value of 0.1 ng/ml. Likewise, sensitivity of βhCG was computed as the percentage of rats over the cut-off of 0.5 IU/l in the Ad-NIS-CG group. Each sensitivity estimate per parameter above was calculated at the three measurement days and over all 3 days.
We further evaluated each of the three parameters’ ability to distinguish rats that were perfused with the virus versus those that were not at various days using logistic regression. To predict those with a specific virus, we used the corresponding parameter measurement on each and then over all three days. The c-statistic from the logistic regression, a measure gauging the model’s discriminatory ability, was reported for each parameter at the study time points. In addition, standard errors for the c-statistic were calculated using the DeLong method allowing formal comparisons between pairs of three parameter c-statistics. p-Values were obtained assuming the three c-statistic estimates were normally distributed, and thus that the difference between any two could also be considered normal. A significance level of 0.05 was used for all analysis.
3 Results
3.1 SPECT/CT image intensity quantification
All animals underwent SPECT/CT sequential imaging after tail vein injection of 1000 μCi 123I on POD 5, 10 and 15. Recipients were sacrificed following the imaging protocol on day 15, transplanted heart weight was measured and intensity of images obtained from each transplanted heart was quantified as described above.
Significantly higher image intensity was noted in the hearts perfused with Ad-NIS (1.1 ± 0.2; 0.9 ± 0.07), Ad-NIS-CEA (1.2 ± 0.3; 0.9 ± 0.1) and Ad-NIS-CG (1.1 ± 0.1; 0.9 ± 0.1) compared with the UW group (0.44 ± 0.03; 0.47 ± 0.06) on POD 5 and 10, respectively (%ID/g; mean ± SD) (p < 0.05). Image intensity at days 5 and 10 was higher than at day 15 in the hearts transduced with Ad-NIS, Ad-NIS-CEA and Ad-NIS-CG (Table 1 ) while UW group image intensity did not differ on sequential imaging over time. A peak in image intensity on day 5 followed by a fall to background level by day 15 was noted and reflects the serotype 5 recombinant adenovirus kinetics in the transplanted hearts (Fig. 1 ).
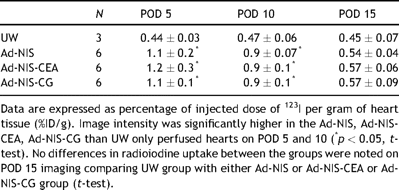
Image intensity quantification on POD 5, 10 and 15 following gene transfer or UW only perfusion
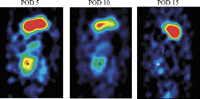
Micro-SPECT antero–posterior view of the abdomen of the same animal showing the transplanted heart on POD 5 (left), 10 (middle) and 15 (right) after Ad-NIS perfusion. The hot spot corresponds to the position of the transplanted heart (*). The hot spot above the transplanted heart corresponds to the 123I concentration in the stomach due to the inherent presence of NIS in the gastric mucosa. The regions of interest were defined manually around the heart shadows on the reconstructed axial tomographic images in all sections in which the heart was visualized, and quantification performed on the co-registered micro-SPECT images. This ensured accurate coregistration of the axial CT images with the micro-SPECT maps.
3.2 Soluble marker hCEA and βhCG serum quantification
Serum quantification of soluble markers hCEA and βhCG was performed on blood collected from all the recipients on POD 5, 10 and 15 before each 123I injection and micro-SPECT/CT imaging.
In the Ad-NIS-CEA group mean ± standard deviation serum levels of hCEA were 4.4 ± 6.0, 0.9 ± 1.1 ng/ml on POD 5 and 10, respectively. On POD 15 hCEA serum levels were below the threshold (0.1 ng/ml) for all six animals. As for image intensity a peak level was noted on POD 5 followed by a progressive decrease level by day 15. hCEA levels were significantly higher in Ad-NIS-CEA group compared to the other three groups on days 5 and 10. No difference was found between the groups on day 15. hCEA was undetectable in all the samples obtained from UW, Ad-NIS and Ad-NIS-CG perfused hearts.
Differently from the trend observed for hCEA levels in Ad-NIS-CEA group, in the hearts perfused with Ad-NIS-CG, serum levels of βhCG were significantly higher (7.8 ± 6.2 IU/l) only on POD 5 respect to POD 10 and 15 (p < 0.05). On POD 10 and 15 βhCG serum levels were constantly below the threshold (0.5 IU/l). In the hearts perfused with UW only, Ad-NIS or Ad-NIS-CEA, βhCG was undetectable in all the serum samples analyzed. Soluble marker serum levels are listed in Table 2 .
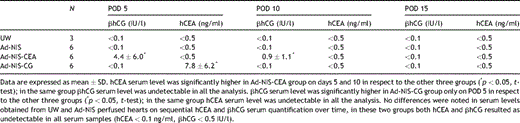
Soluble marker hCEA and βhCG serum level quantification on PD 5, 10 and 15 following gene transfer or UW only perfusion
3.3 Anti-hCEA and anti-βhCG antibody screening
Anti-hCEA antibodies were detected on serum samples from Ad-NIS-CEA rats only on POD 15 while they resulted negative on POD 5 and 10 (Fig. 2A ). Serum samples collected from Ad-NIS-CG rats on POD 5 contained no detectable anti-βhCG antibodies. In this group, different from Ad-NIS-CEA group, on POD 10 and 15 significantly high-titers of anti-βhCG antibodies were detected (Fig. 2B). These findings explain the washout of serum hCEA and βhCG noticed on POD 15 and after POD 5 respectively.
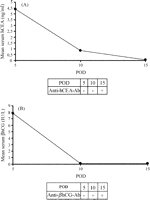
Mean serum levels of hCEA (A) and βhCG (B) on POD 5, 10 and 15. The presence or absence of anti-hCEA or anti-βhCG antibodies in the serum samples are as indicated.
3.4 Correlation between micro-SPECT imaging and soluble markers quantification
Image intensities of micro-SPECT images expressed as %ID/g were compared with hCEA and βhCG serum levels at POD 5, 10 and 15. The analysis was performed comparing %ID/g versus hCEA levels in Ad-NIS-CEA perfused hearts and %ID/g versus βhCG levels in hearts perfused with Ad-NIS-CG. Serum levels of hCEA and βhCG correlated well with image intensities on POD 5, R2 = 0.99 with p < 0.001 and R2 = 0.96 with p = 0.001 respectively (Fig. 3A and B ). On POD 10 a strong correlation was found between %ID/g and hCEA levels (R2 = 0.88, p = 0.006) as shown in Fig. 4 . The %ID/g and βhCG serum levels did not correlate on the same POD because of βhCG serum levels constantly below the detection limit. No correlation was found between %ID/g and both the soluble markers levels on POD 15 (data not shown).
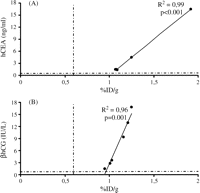
Comparison of image intensity %ID/g with hCEA (A) or βhCG (B) serum levels on POD 5. Dashed lines represent the thresholds of %ID/g in both A and B, hCEA in A and βhCG in B. After 5 days from ex vivo heart perfusion with Ad-NIS-CEA or Ad-NIS-CG image intensities and soluble markers serum quantifications correlate very well (A: %ID/g vs hCEA R2 = 0.99, p < 0.001 and B: %ID/g vs βhCG R2 = 0.96, p = 0.001).
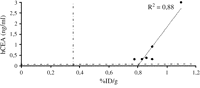
Comparison of image intensity %ID/g with hCEA serum levels on POD 10. Dashed lines represent %ID/g and hCEA thresholds. After 10 days from ex-vivo Ad-NIS-CEA heart perfusion %ID/g still correlates well with hCEA serum levels (R2 = 0.88, p = 0.006). No correlation was found on the same postoperative day between image intensities and βhCG serum levels (from POD 10 βhCG levels were constantly below the threshold).
This result suggests that serum levels of hCEA and βhCG increased only in animals showing high cardiac 123I uptake, but not in those with lower uptake. Above this threshold (0.6 %ID/g), image intensities correlated well with serum levels of hCEA and βhCG. The threshold value for image intensity (%ID/g) was estimated from the UW-only perfused-hearts data pooled across the three time points (POD 5, 10 and 15), which represented the range of baseline amount. Since this cut-off served to differentiate baseline versus virus-induced %ID/g, the maximum value from the baseline data plus one standard deviation was chosen as the threshold value (0.6 %ID/g).
3.5 Sensitivity and logistic analysis
The sensitivity of %ID/g computed at day 5, 10 and 15 was 100, 100 and 16.7%, respectively (Table 3(A) ). In a logistic regression model predicting any of the three Ad-NIS, Ad-NIS-CEA and Ad-NIS-CG treatment groups versus UW group using %ID/g value, the c-statistic was 1.0, 1.0 and 0.90, respectively. Pooling across all 3 days, the sensitivity was 72.2%, and the corresponding logistic analysis yielded a c-statistic of 0.97 (95% CI: 0.92, 1.00), indicating very strong discriminatory ability.
(A) Sensitivity of %ID/g, hCEA (ng/ml) and βhCG (IU/l) computed at POD 5, 10 and 15. (B) Values represent the c-statistics in a logistic regression model predicting any of the three Ad-NIS, Ad-NIS-CEA and Ad-NIS-CG treatment groups versus UW group
For hCEA serum, the sensitivity was 100% at days 5 and 10 and 0% at day 15. When pooling days together, the sensitivity was 66.7% and the c-statistic was 0.83 (95% CI: 0.72, 0.95). As for the other serum parameter βhCG serum level, the sensitivity at day 5 was 100% and then it dropped down to 0% at day 10 and 15. Essentially, pooling across days, the overall sensitivity of βhCG serum levels was 33.3% and the corresponding c-statistic was 0.67 (95% CI: 0.56, 0.78).
To compare the performance of the three parameters, we compared the c-statistics from the pooled data. Specifically, we tested whether the c-statistic for the %ID/g parameter was significantly higher than that of the hCEA or the βhCG serum level parameters. The ability of %ID/g of discriminating those induced with Ad-NIS from those not was significantly higher than the discriminatory ability of hCEA among those with and without Ad-NIS-hCEA and of βhCG among those with and without Ad-NIS-βhCG (p-value = 0.03 and <0.001, respectively) (Table 3(B)).
4 Discussion
Recent improvements in experimental research of gene transfer suggest that gene therapy may become a promising choice for the treatment of rejection [12]. The success of this therapy depends on the efficiency of gene transfer with the use of a nonimmunogenic and durable vector, such as adeno-associated virus (AAV).
In this study the heterotopic hearts perfused with a solution containing either Ad-NIS, Ad-NIS-CEA or Ad-NIS-CG presented a significantly higher uptake of radioiodine with respect to the control group (UW only), 5 and 10 days after the ex vivo perfusion. Except for the last group all transplanted hearts showed an image intensity peak on POD 5 with a radioiodine uptake that was still significantly higher on POD 10 than in the control group. The percentage of injected dose per gram returned to the baseline level by day 15 revealing no difference with respect to the controls. This trend was expected according to the kinetics of adenovirus serotype 5.
It would be tempting to state that hNIS gene is more sensitive as a reporter gene than hCEA or βhCG, which code for soluble marker peptides. Serum levels of soluble markers peptide increased in animals showing increased cardiac I123 uptake, but not in those with decreased uptake. However, the threshold value for image intensity (%ID/g) estimated from the UW group represents only arbitrarily the range of a baseline detection level. Again, strong correlation between image intensity and serum level of soluble markers was noted for both hCEA and βhCG on POD 5 when, according to the adenovirus 5 kinetics, the maximum gene expression is expected. Only hCEA levels were highly correlated with the radioiodine uptakes after 10 days post-transplantation while βhCG resulted as undetectable in all the samples. This result can be explained by the presence in serum of a high-titer of anti-hCEA and anti-βhCG antibodies after POD 10 and 5 respectively. In addition the small number of grafts in our study in each group obviously limits final conclusions concerning the sensitivity of these markers.
Gene therapy is a very promising type of treatment for many different diseases. In theory, gene therapy can repair the cause of genetic disorders on the most basal level and thereby be very specific and effective. By selectively introducing genes that encode for so called suicide proteins, even cancer can be treated with gene therapy. It has become clear that the success of the therapy is highly dependent on the efficiency of gene transfer with the use of a non-immunogenic and durable vector. It is also mandatory to define a non-invasive method to monitor gene transduction.
Ideally, in any gene therapy study, we should know the number, distribution and fate of the genetically modified cells, and the kinetics of production of the therapeutic protein over time. Unfortunately, for most transgenes this type of information is very difficult to obtain. Mapping the distribution of genetically modified cells typically requires euthanasia and thorough post-mortem analysis of all tissues using in situ hybridization, immunohistochemical analysis, or determination of enzymatic activity in tissue extracts. Non-invasive imaging has recently been used to map the distribution of transgene expression in one or two special cases where the transgene product can be used to track a positron-emitting radionuclide at its site of expression [13].
Equally problematic is the need for non-invasive methods to monitor the profile of gene expression over time. Expression of cell-associated transgenes can be monitored by immunohistochemical or other analysis of tissue biopsies obtained from the site of gene transfer [14–16]. However, for most vector systems and most routes of administration, gene transfer within the target site is non-uniform. Tissue sampling is therefore an unsatisfactory method to assess the efficiency of gene delivery because the biopsy that is subjected to analysis is essentially a random sample from an area of non-uniform gene delivery. Also, there is a limit to the number of times that a given tissue can be biopsied, and for certain tissues, such as the heart, tissue biopsy is associated with a high risk of fatality.
Even for secreted transgene products, the monitoring of expression can be fraught with problems. First, the secreted protein may be retained in the tissues through a heparin-binding or matrix attachment region or through its interaction with its naturally occurring receptor. Thus, human cardiovascular gene therapy studies, employing vector coding for vascular endothelial growth factor, were associated with transient or undetectable circulating levels of the protein [17–19]. Second, the soluble protein encoded by the transgene may be indistinguishable from its naturally occurring counterpart. This is a problem in cancer gene therapy studies where immunostimulatory cytokines, such as IL-2, GMCSF and IL-12 expressed from a transgene delivered in the tumor microenvironment, cannot be distinguished from the same cytokines expressed from activated macrophages and lymphocytes that are part of an inflammatory response against the vector. Third, for certain proteins there may be no suitable assay available for sensitive detection. Finally, the soluble protein may be the target of a humoral immune response that causes a precipitous fall in the circulating level giving the misleading impression that its production is attenuated.
Our data demonstrate that hNIS is an excellent reporter gene for the transplanted heart. The expression level of hNIS can be accurately and non-invasively monitored by serial radioisotopic SPECT imaging. In addition hNIS gene transfer permits sequential real-time detection and quantification of reporter gene expression in the transplanted heart. We also demonstrated that there is a very high concordance between imaging and soluble marker peptides. Thus, the expression level of one gene can be inferred from the measured expression level of the other.
In myocardium NIS protein does not interact with the cell biochemistry since iodide is not metabolized. Despite the concern in the use of NIS because of the possible membrane potential effects in the transfected myocytes, in this study all animals were clinically stable throughout the observation period with a preserved palpation score (6/6). Lee et al. [8] in their study demonstrated, in fact, that myocardial NIS gene imaging does not cause significant myocardial injury or perturbed cardiac function other than mild effects due to adenoviral vector-associated host response. In addition the use of a species-specific isoform such as hNIS for humans may help to circumvent any problem caused by the immunogenicity of transgene products.
Adenovirus elicits a strong host immune response; this interferes imaging of reporter gene expression over about ten to fourteen days after adenovirus transfection. Transient expression due to immunogenic reaction is a very well known limitation of this vector. Despite these limits we choose to use a recombinant adenoviral vector, with a deleted E1 region to prevent replication in vivo, because of the easy production, the long-term experience and the high efficiency obtained with this vector [10,20–22].
The aim of this study was to demonstrate the usefulness of hNIS as reporter gene for SPECT/CT imaging independently of the vector used for transfer gene. Moreover, the use of this technique with a dual-gene vector appears to allow accurate assessment of the level of myocardial expression of a second gene of interest.
The results obtained lead to future applications of the same reporter gene encoded into less immunogenic and more durable vectors like recombinant AAV [23]. These vectors could ensure, for a long period, an adequate time-course monitoring of transplanted cells after ex vivo transduction. Recently we have made AAV vectors coding for hNIS, that unlike adenovirus vectors do not encode viral proteins; in this way the transduced cells are not immunogenic. Preliminary in vivo data are looking promising.
Finally, the use of ex vivo perfusion technique is feasible in the large animal model [24]. This method was not used in this study due to constraints with the production of large quantities of the vector. However, the translation of this technique to humans would be straightforward due to the expertise already gained with SPECT imaging of the heart in clinical studies. The use of SPECT/CT in the transplanted human heart would also facilitate precise quantification of cardiac dimensions and exact sites of gene transfer. Small animal SPECT/CT scanners are limited in their resolution. Soft tissue and bowel shadows in the abdomen in this heterotopic model may have affected definition of transplanted heart margins.
Taken together, our data suggests that hNIS is a most promising reporter gene for the transplanted heart. The expression level of hNIS may be monitored non-invasively by serial radioisotopic SPECT imaging. In addition, hNIS gene transfer permits sequential real-time detection and quantification of reporter gene expression in the transplanted heart.
Acknowledgements
We wish to acknowledge the contributions of Dr Kent Bailey and Brian Lahr for statistical analysis, Teresa Decklever for imaging assistance and Karen Schumacher for writing assistance.
References
Author notes
Presented at the 21st Annual Meeting of the European Association for Cardio-thoracic Surgery, Geneva, Switzerland, September 16–19, 2007.
This research is supported by NIH Grant HL66598 and the William J. von Liebig Foundation.