-
PDF
- Split View
-
Views
-
Cite
Cite
Ron Ben Abraham, Menachem Matza, Sylvia Marmor, Valery Rudick, Inna Frolkis, Itzhak Shapira, Avi A. Weinbroum, Electromechanical impairment of human auricle and rat myocardial strip subjected to exogenous oxidative stress, European Journal of Cardio-Thoracic Surgery, Volume 23, Issue 1, January 2003, Pages 66–73, https://doi.org/10.1016/S1010-7940(02)00729-7
- Share Icon Share
Abstract
Objective: Animal myocardial dysfunction induced by remote ischemia-reperfusion (IR) was shown to be partly accomplished via a direct effect of the pro-oxidant xanthine oxidase (XO). This direct remote effect was not tested in humans. We now assessed the performance of human auricles in the presence of solutions containing XO and/or allopurinol and compared them to those of rat myocardial strips. Methods: Human and rat specimens (n=64) were separately exposed for 2 h to Krebs–Henseleit solution that either (1) exited from rat livers that were earlier perfused for 2 h (control-human or control-rat), (2) exited from livers that were earlier made ischemic for 2 h (IR-human, IR-rat), (3) contained xanthine (X) 3.8 μM+XO 3 mU ml−1 (X+XO-human, X+XO-rat), or (4) exited from post 2 h-ischemic livers and contained 100 μM allopurinol (human or rat IR+allopurinol groups). Results: Unlike the unchanged electromechanical performance in the control and IR+allopurinol auricles and strips, the rates of contraction, maximal force of contraction and working index of either preparation were reduced by 75–98% (P<0.01) when exposed to the IR reperfusate or to the X+XO-enriched Krebs. The basal amplitudes of contraction in these four latter groups increased twofold (P<0.01). XO activity was similarly low in the control and in the IR+allopurinol groups, but four- to 45-fold (P<0.001) higher in the IR and the X+XO groups, both in the rat and human organs. The reduced glutathione was reduced by ∼50% (P<0.01) in either preparation in the IR and the X+XO groups compared to the control and IR+allopurinol groups. Conclusions: Remotely and exogenously originated oxidative burst directly induces electromechanical dysfunction and disrupts oxidant/antioxidant balance in human auricles as it does in the rat myocardial strip.
1 Introduction
Ischemia and subsequent reperfusion (IR) are associated with thrombolytic therapy, organ transplantation, coronary angioplasty, aortic cross clamping and cardiopulmonary bypass. These events cause both localized and systemic injury, leading to functional loss [1]. The growing number of surgical procedures in which such conditions may occur and their untoward consequences underline the clinical importance of this phenomenon [2]. Arrhythmia, impairment of the myocardial force of contraction and possible cell death characterize myocardial injury both experimentally and clinically. The various pathophysiological alterations that may occur during this process have been largely attributed to reactive oxygen species (ROS) [1,2] which are abundant in the effluent that exits the organ(s) or area(s) that had been earlier subjected to IR.
In our previous investigations, we had used a rat isolated perfused double-organ model (liver and heart) and documented a reduction in myocardial velocity of contraction and relaxation when the heart was exposed to liver post-ischemia effluent [1], events that were abrogated by the pre- and intra-experimental administration of allopurinol and other antioxidants [1]. The effects of ROS-generating systems on the myocardium have been described long ago, e.g. when subjecting the myocardium itself to IR [3–5]. These and other data have led to the conclusion that xanthine oxidase (XO) and its generated ROS have a direct and primary detrimental role not only when the heart is made ischemic and then reperfused, but also in rat remote reperfusion-induced cardiac dysfunction as well. In view of the major roles played by oxidative stress, organ injury and the antioxidant mechanism of tissue protection, it is surprising that the direct influence of XO- and ROS-generated injury in one area on the normal human heart has not been described in the literature. To contribute more knowledge on the effect of such event in one area of the body on a part of the human heart, we used our double-organ model to establish the effects of a post-ischemic liver reperfusate or a Xanthine (X)+XO-enriched perfusion solution on the performance of human auricles in the presence or absence of the oxidoreductase inhibitor, allopurinol. We also compared these effects with those generated in rat heart strips.
2 Materials and methods
All the animal experiments were carried out in accordance with guidelines established by the Institutional Animal Care and Use Committee at the Tel Aviv Medical Center and the Tel Aviv University. All the human experiments were approved by the Institutional Committee for Human Studies and each participant signed a Helsinki-approved informed consent.
2.1 Human auricle and rat myocardial strip preparation
The auricles used in the current study were obtained from patients classified as ASA I–III and New York Heart Association class I–II and who underwent elective aortocoronary revascularization (open heart) surgery for the first time. The same surgeon performed all the human operations (M.M.) and the same anesthetist administered anesthesia (A.A.W.). No patient had suffered from a previous myocardial infarction or had a history of right ventricle dysfunction, pulmonary hypertension, valve disease or atrial fibrillation. Patients with diabetes mellitus were also excluded from the study. All the subjects were hemodynamically stable (mean arterial blood pressure >70 mmHg), did not exhibit atrial dysrhythmia and had not experienced angina for >96 h prior to surgery. Their central venous pressure was ≤10 mmHg upon the insertion of a central venous line. None of the patients had received digoxin, calcium- or beta-blockers.
All auricles were removed from the patients before the starting of the cardio-pulmonary bypass; their mean age was 66±10 years (range 45–78) and they had a left ventricular ejection fraction of 52±13%, both similarly distributed among the groups (data not shown). During transportation from the operating room to the laboratory, the acquired auricles were kept in warm, oxygenated, hemoglobin-free, modified Krebs–Henseleit solution (Krebs), as described elsewhere [6]. Once in the laboratory, they were placed in fresh oxygenated Krebs solution at ambient temperature. Each auricle was carefully dissected into a strip 15–25 mm in length and 4–5 mm in diameter before being appended within the organ chamber (see subsequently).
Adult male Wistar rats (320–400 g) were anesthetized by intraperitoneal sodium pentobarbital (50 mg kg−1) and were also given 300 IU of heparin sulfate. After thoracotomy, the animal's heart was removed and placed immediately within fresh, oxygenated, ice-cold Krebs solution. A myocardial strip 10–13 mm long and 2–3 mm wide was then prepared as previously described [6].
2.2 Experimental model
Each of the preparations was placed within a 50-ml chamber containing oxygen-enriched (0.5 l min−1) Krebs at 37°C. One end of the strip was attached to a force transducer (FT-03 Grass instruments, Quincy, MA, USA) by a thin silk thread, while the other end was pinned to a hook in the tissue bath. The strips were left for 15 min or more to spontaneously recover their isometric tension, after which they were gradually stretched to a resting force of 1 g, which had been determined previously in our laboratory and by Cleveland et al. [7] to be an optimal length–tension relation for both groups of strips. They were then allowed to re-equilibrate for as long as needed (usually 15–30 min). The strips' bathing solution was refreshed at 20-min intervals throughout stabilization.
At ∼30 min following their isolation, both the human and animal strips resumed spontaneous electrical activity which was accompanied by contractile activity as well (see subsequently). For those cases in which this did not occur within this initial equilibration period, epinephrine in increasing concentrations (0.004–0.04 mM) was added to the tissue bath until the force of contraction reached a steady plateau value >0.75 g and a beating rate ≥40 min−1 in human strips and ≥80 beats min−1 in the rat strips. A negative effect of the drug on a strip excluded it from the study. The Krebs solution was then refreshed and the muscle was left to beat undisturbed until the double-organ experiment was started (see subsequently). The auricles and the rat strips were never subjected to ischemic conditions.
We used rat strips rather than the Langendorff non-working heart model in the current study [1] because the current model better suited for a comparison of rat electromechanical events with those of human auricles. Also, the 120-min duration of exposure of the specimens was chosen to unequivocally determine even the subtle changes in the degree of injury as well as a possible recovery of the specimens from such events [1].
2.3 Isolated liver preparation
Livers from separate rats were isolated and prepared as previously described [1]. Briefly, large bore cannulae (14-gauge) were placed in the portal vein and the supra-hepatic inferior vena cava, and they served as the respective ports for flow entrance and exit, for aliquot sampling and pressure measurements. The liver was then extirpated and placed in an environmental chamber where temperature (37.5°C) and moisture were kept constant. The liver was perfused with Krebs at a rate of 3–4 ml min−1 g−1 ideal liver weight and as required to maintain an exiting normal pH and pCO2. The perfusate was maintained at a constant temperature (37°C) and equilibrated with 95% O2/5% CO2 to achieve a pre-organ pO2 ≥350 mmHg, pCO2 34–38 mmHg, and pH between 7.34 and 7.44.
The isolated livers were allowed to stabilize for 30 min to clean out the microcirculation from the blood and debris and to reestablish normal circulation; they were then either perfused or made globally ischemic (no flow) for 2 h. At the end of either procedure, the baths were connected to the liver outflow site and the double-organ experiment was started.
2.4 X+XO-enriched Krebs solution
This solution, which was used in two specific experimental groups (see subsequently), consisted of Krebs containing 3.8 μM X+3 mU ml−1 XO. These concentrations had been previously detected in the IR liver exiting reperfusate [1]; much higher exogenously added concentrations had been used previously to inflict oxidative damage upon tissues [8].
2.5 Experimental protocol
Rat or human isolated myocardial preparations (n=8 group−1) were exposed to different solutions for a duration of 120 min. The different groups and various reperfusates are listed in Table 1 .
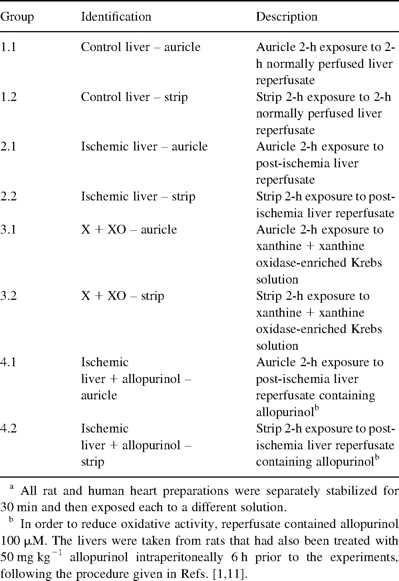
2.6 Evaluation of myocardial performance, biochemistry of the system and histopathological analysis
Electrical activity of the auricles and strips and the spontaneous force of contraction were continuously recorded with an external Harvard® pulse generator/sensor (Edenbridge, Kent, England) and by the above-mentioned force transducer, respectively, both having been logged onto a physiological recorder (Grass Model 7 D polygraph, Grass Instruments Co., Quincy, MA, USA) connected to a hemodynamic monitor (CS3™, Datex-Ohmeda®, Helsinki, Finland). Contractility was measured in terms of the developed force (mmHg). The tested parameters also included (1) basal amplitude of contraction expressed by the diastolic-generated tension, (2) maximal amplitude of contraction expressed by the systolic-generated force, and (3) work index, expressed by the rate of contraction multiplied by the maximal force of contraction. Cleveland et al. [9] had established the basal and maximal amplitudes of contraction as being acceptable modes of evaluating changes in relaxation and contraction of these and similar myocardial specimens.
Biochemical analyses were performed on aliquots from the bath solutions six times during the stabilization phase of the strips and auricles and every 30 min during the exposure to the various solutions, as well as on the organs' tissues after the end of the experiments. Total XO activity (the reduced and oxidized enzyme forms) both in the circulation and in the organs' tissues was analyzed following the procedure of Hashimoto [10] and modifications of it [1]. Reduced glutathione (GSH) content in the myocardial preparations was assessed using a specific assay kit (Calbiochem 354102, San Diego, CA, USA). All analyses were performed in duplicates within 24-h from the completion of experiments.
After termination of the experiments, all auricles and strips were analyzed by a protocol-blinded pathologist (S.M.). The organ biopsies were fixed and preserved in formalin. Hematoxylin and eosin staining for histological examination under light microscopy was used to determine morphological damage of the tissue. Peroxidase and antiperoxidase stain were also applied.
2.7 Statistics
The analyses were performed at the Statistical Laboratory of the School of Mathematics at the Tel Aviv University using the SPSS Release for Windows, Version 9 (1999, USA). The data variables are expressed as means±standard deviation (SD). Comparison of each parameter between two groups at a time (e.g. group 1.1 vs. 2.1) was carried out by ANOVA with repeated measures. When the initial results were different, analyses of co-variants (ANCOVA) was applied to further interpret the data. Each analysis used six baseline values that were averaged to one value, which later was used as a covariate to be compared with its comparable group. The total XO+XDH and GSH values in the 90-min Krebs aliquots and in the tissues were compared using the t-test. Differences in the results of all the tests were considered as being significant when the alpha value was ≤0.05.
3 Results
3.1 Double-organ data
Following their isolation and preparation, seven (22%) of the rat ventricle strips and eight (25%) of the human auricles failed to generate adequate contractile force or to sustain the pre-established rate of contraction even after the pharmacological (epinephrine) resuscitation; they were replaced by other samples. The average time from the auricles' removal until they were mounted within the organ chamber was 5±2 min. The time necessary for the strips of either rat or human origin to successfully recuperate the minimal and constant rate and force of contraction ranged between 14 and 27 min (22±7). Ten percent and 7% of the rat and human viable strips, respectively, required an initial stimulant jolt (data not shown). There were no intra-group differences between the preparations that required epinephrine to initially recover and those that resumed electromechanical function spontaneously (data not shown); the data for each group are, therefore, presented as an average of all replicates in the group.
There were only a few episodes of arrhythmia and suboptimal force of contraction during the initial recovery phase of the preparations and these disappeared after a negligible period of time.
3.2 Biochemistry of the double-organ system
All the biochemical values of the preparations during stabilization were similar among the groups (data not shown). The total XO activity is reported in Table 2 . The activity increased by ∼50-fold (P<0.01) in the bath solutions and by four- to sevenfold in the tissues of the ischemia and the X+XO groups (groups 2.1, 2.2, 3.1 and 3.2) compared to the controls (groups 1.1 and 1.2). The allopurinol-treated groups (groups 4.1 and 4.2) showed a substantial reduction of XO, almost to the level of the control groups' activity, both in the bath solutions and in the tissues of the auricles and the rat strips.
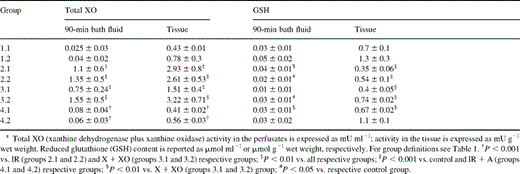
The GSH levels in the bath solutions and in the tissues are also reported in Table 2. There was a significant (P<0.01) reduction in the level of GSH in the solutions and in the tissues of the specimens in the ischemia groups (groups 2.1 and 2.2) compared to the controls'. The GSH pool was also depleted in the X+XO groups (groups 3.1 and 3.2) compared to the controls, although to a lesser extent than in the respective IR groups. The IR+allopurinol groups (groups 4.1 and 4.2) maintained levels of GSH activity that were very similar to those of the controls.
3.3 Performance of myocardial preparations: rate and force of contraction
Both control preparations (groups 1.1 and 1.2) exhibited a regular electromechanical activity (rate and force of contraction) during stabilization (Figs. 1 and 2 ). Their exposure to the IR perfusate (groups 2.1 and 2.2) caused the rate of contraction to decline immediately and sharply (Fig. 1). This significant (P<0.01) negative chronotropic effect was followed 20–40 s later by a reduction in the force of contraction (Fig. 2). Similar effects were also observed in the two X+XO-enriched Krebs groups (groups 3.1 and 3.2).
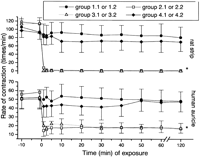
Changes in the rate of contraction of the auricles and the strips. For group definitions see Table 1. *P<0.01 vs. control (groups 1.1 and 1.2) and ischemia+allopurinol (groups 4.1 and 4.2).
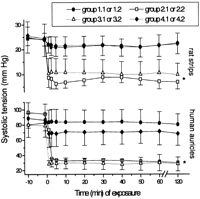
Changes in the maximal amplitude of contraction of the organs. For group definitions see Table 1. *P<0.01 vs. control (groups 1.1 and 1.2) and ischemia+allopurinol (groups 4.1 and 4.2).
Upon the exposure of the two types of muscles to the IR+allopurinol solutions (groups 4.1 and 4.2), a brief and slight decrement appeared in both species' electromechanical performances, which then returned to almost stabilization values and remained unchanged from the controls thereafter (Figs. 1 and 2).
3.4 Performance of myocardial preparations: amplitudes and work index
Because these parameters were of similar values among all groups at baseline, their data are presented as a percentage of baseline values (Table 3 ). At the same time the groups 2–3 demonstrated the above-mentioned electromechanical disturbances, their basal amplitudes (expressed by the extent of diastolic tension) increased while the maximal amplitudes of contraction (expressed as the extent of systolic tension) decreased significantly (P<0.01) from those of the controls and their own baseline levels. This narrowing in the amplitudes of the generated tensions was, however, of a similar magnitude in the two species. The IR (groups 2.1 and 2.2) and the X+XO (groups 3.1 and 3.2) groups also showed an 86–100% loss in their work indexes starting within 2 min from their exposure to the various solutions (Table 3). The functional collapse was more severe in the rat than in the human preparations.
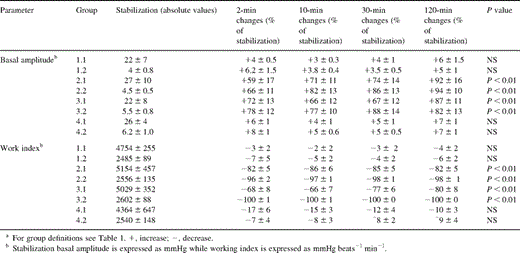
The exposure of the organs to allopurinol-added ischemic reperfusates (groups 4.1 and 4.2) was associated with a mild and brief deviation from the control values of the basal and maximal amplitudes, as well as those of their work index (Table 3). The values then remained unchanged throughout the experimental period, reiterating the above-mentioned allopurinol's protective effect on the rate and force of contraction of the strips and the auricles.
3.5 Histopathological analysis
The histopathological evaluation of the human muscle preparations indicated no anatomical changes in any of the specimens. There were, however, myocytolysis in the subendocardium. These were identified by central vacuolization surrounded by pink-staining sarcoplasm, which was sometimes limited to a thin rim of tissue at the cell periphery (Fig. 3 ). These changes also appeared in the immunohistochemical stain of the myoglobin. The vacuoli, however, failed to stain in contrast to the remaining sarcoplasm of the myocardial fibers.
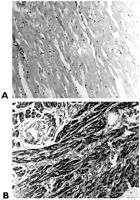
Histopathological sections and staining of the human auricle. (A) Control (group 1.1) myocardium: normal smooth muscle without changes in the myocytes (hematoxylin & eosin, original magnification; ×10); (B) X+OX (group 3.1) myocardium is intensely stained by myoglobin. Cytoplasmic vacuoli are observed in the myocytes that were sectioned perpendicularly (peroxidase–antiperoxidase stain, original magnification; ×20).
4 Discussion
Our observations with human tissue are preliminary but contribute some important new insight into the direct effect of oxidative stress on the human auricle. The damage induced to the human myocardium and the protection afforded by allopurinol to this simulated remote IR damage has not been reported previously. All specimens lost their normal electrical and mechanical function when exposed to the reperfusate that either exited from the ischemic-reperfused liver or to Krebs+X+XO, both solutions containing high concentrations of XO, the generator of ROS [1,2,5]. The present data of the human specimens support our previous contention that remote organ IR can induce myocardial reperfusion dysfunction, and that such a toxic process is humorally mediated [1]. A direct cause-and-effect relationship – one of the principal goals of our current study and yet unexplored in human auricles – was also established: the XO-induced electromechanical deterioration was prevented by its oxidoreductase inhibitor, allopurinol. Interestingly, our results also demonstrated a similar functional deterioration occurring in the rat heart muscle strips and human auricles following the same toxic exposure.
Our present hypothesis that the human heart could directly react to distant IR events similarly to the rat heart follows not only our previous reports of a direct relationship between remote IR and various rat organ depression [1,11,12], but also earlier comparisons of damage among species by Cleveland et al. [7], an investigational report regarding human heart reperfusion injury [13] and a description of various modalities of protection in different species [1,14], as well as earlier documentations of IR heart dysfunction [3]. Our present findings thus add to the previous studies showing that both human and animal myocardium dysfunction that may occur after remote site IR (e.g. organ transplantation or aortic cross clamping) could be of a similar toxic pathway [1,2] and might therefore involve similar solutions. While demonstrating that the human auricle was functionally depressed exclusively via the XO-ROS humoral pathway, our results also indicate, presumably for the first time, that an XO-generated oxidative stress would first interfere with the physiological rhythmicity of the human auricle, and only afterwards would it lead to a depressed force of contraction. This is possibly related to the ‘Bowditch staircase effect’: the slower the heartbeats are the weaker is the force of contraction [15].
The reperfusion of an ischemic area results in the formation of toxic ROS, including superoxide anions (O2−), hydroxyl radicals (OH−), hypochlorous acid (HOCl−), hydrogen peroxide (H2O2), and nitric oxide-derived peroxynitrite. Exogenous XO added to the isolated perfused rabbit heart was shown to produce a concentration-dependent decrease in left ventricular contractility similar to the effects of ROS and preventable by superoxide dismutase [16]. Subjecting dogs to similar protocols, Prasad et al. [16] also detected a XO-generated marked decrease in heart rate, left ventricular systolic and end-diastolic pressure, ±dP dt−1MAX. Gupta and Singal [17] demonstrated an X+XO association with a decline in peak-developed force and dF dt−1 within 5 min from the perfusion of the Langendorff preparation, and complete contractile failure was recorded at 20 min. Finally, a semi-quantitative morphometry documented both myocardial and vascular changes 10 min after starting X+XO reperfusion. These data strongly support our contention of an X+XO-mediated decrease in heart rate and myocardial contractility depression, and are very much compatible with our present findings on human auricles. Noteworthy, the reported lower XO activity in the human heart than that in the rat [18], for example, does not weaken the significance of our results, since injury was afflicted to either specimen. The high levels of XO activity in the tissues after the injury do not necessarily correlate to their physiological XO content: as we have shown earlier [1,11], when the tissue is injured, the exogenous XO enters the tissue and additively engineers the formation of XO and ROS in it.
The decreased rate of contraction that preceded the reduction in the force of contraction could be related to a vulnerability of the myocardium cells' automaticity in the presence of ROS activity. ROS toxicity might interfere with the ATP-dependent energetic process [19], which may result in external–internal electrolyte disturbances and Na+/K+ pump dysfunction. Damage that was afflicted to various components of the heart by lipid peroxidation [14] and the increased level of the intracellular generation of ROS are other explanations for this phenomenon. Indeed, ROS are potent oxidizing and reducing agents that directly damage cellular membranes by lipid peroxidation [20]. Finally, the histological changes in the injured preparations, compared to the normal controls, represent a direct toxic degradation of structural or functional proteins of the mitochondria, myofilaments and membrane constituents that were exposed to the action of the toxins added to the baths.
Ischemia and subsequent reperfusion are also associated with depletion of the organs' natural antioxidant defense systems, such as the glutathione system [21]. The reduced GSH plays a central role in the cellular defense against ROS, and it traps H2O2 and consequently decreases the production of other highly reactive hydroxyl radicals. It is a natural scavenger of the superoxide anion and protects the protein thiol group, which is essential for protein function and cellular integrity. GSH levels decrease during ischemic insult and during reperfusion, for which GSH levels were shown to reliably index the magnitude of protection vs. injury following such occurrences in several animal models [21,22] and as was herein observed. Our data now indicate GSH's involvement in remote IR-induced human heart damage: the effect of the IR reperfusate and Krebs-X+XO depleted GSH – much more by the former than by the latter – compared to the control. Allopurinol, by diminishing the generation of the oxidative stress, enabled a better preservation and a lesser consumption of GSH even in the human tissue where XO is less abundant than in the rat [18]. Comparatively, the dissimilar content of GSH in the tissue of the human auricles and the rat strips could be reflective of the lower levels of this enzyme in human tissues compared to other mammals [23]. Given the limitation of the specific double-organ experimental model used in the current study, however, the ‘preferential’ site of activity of allopurinol was on the liver because this organ can metabolize XO to high activity compared to the heart, especially in humans. Since XO and ROS are generated within the hepatic tissue and within the circulation upon reperfusion ex-novo, the low activity of XO in the post-liver circulation and in the strips when allopurinol was added serves to confirm this line of thinking [11].
We do not intend to directly extrapolate from this ex vivo double-organ model onto in vivo conditions where part of the ROS might well be scavenged while circulating in the blood and at the same time blood-circulating elements (e.g. neutrophils) may accentuate the oxidative burst. Nevertheless, the likelihood that remote organ IR (e.g. limb tourniquet, aorta cross clamping, low perfusion state) can directly induce myocardial dysfunction through a pro-oxidative humoral process is of a clinical importance, it being a hemodynamic threat [24,25]. From a clinical standpoint, upon the appearance of myocardial reperfusion injury, especially when associated with low perfusion state, it would seem wise to consider in the first place maintaining heart rate constant, which might attenuate subsequent loss of cardiac force of contraction, while, at the same time, applying a selective antioxidant treatment to the heart, as suggested for the lung [21] or the vasculature [1], to further prevent hemodynamic collapse. Noteworthy, the relevance of the presented data of XO and possible ROS toxic activity on remote organs is not limited to the auricle, which represents the right side of the heart. It is also applicable to the left side of the heart and, eventually, to other organs, even though the lung is known to provide efficacious albeit limited biochemical and toxicological filtering. As demonstrated in our previous study [11], the lungs were capable of excluding approximately 50% of XO while being perfused by the solution that contained it, which led to a build-up of up to 14-fold activity by the end of the experiment compared to the lung's own XO baseline. However, this process caused the formation of an ARDS-like condition within the lung. The biochemical explanation for such sequelae is manifold: direct generation of oxidants within the intravascular compartment while XO and purine substrate traverse the circulation [26,27] or the binding of XO to the glycosaminoglycans of vascular endothelial cells where it is concentrated and its half-life is prolonged, thus extending the damage throughout the body [28].
Our present demonstration of a direct role of XO in interfering with human auricle function, the protective role of allopurinol, the corresponding GSH levels in the tissue as well as the toxic changes in the preparations, are the first ex vivo report of its kind. The results of this study represent a clinically interesting suggestion that XO and ROS could reach the heart and cause heart injury in vivo. Nevertheless, this contention still requires confirmation by future experimental and clinical studies before pharmacological considerations are undertaken.
We thank Esther Eshkol for editorial assistance. This study was supported in part by the Anonymous Italian Family Fund, Milan, Italy.