-
PDF
- Split View
-
Views
-
Cite
Cite
Huan Xu (徐焕), Qiuying Huang (黄求应), Yongyong Gao (高勇勇), Jia Wu (吴佳), Ali Hassan, Yutong Liu (刘昱彤), IDH knockdown alters foraging behavior in the termite Odontotermes formosanus in different social contexts, Current Zoology, Volume 67, Issue 6, December 2021, Pages 609–620, https://doi.org/10.1093/cz/zoab032
- Share Icon Share
Abstract
Foraging, as an energy-consuming behavior, is very important for colony survival in termites. How energy metabolism related to glucose decomposition and adenosine triphosphate (ATP) production influences foraging behavior in termites is still unclear. Here, we analyzed the change in energy metabolism in the whole organism and brain after silencing the key metabolic gene isocitrate dehydrogenase (IDH) and then investigated its impact on foraging behavior in the subterranean termite Odontotermes formosanus in different social contexts. The IDH gene exhibited higher expression in the abdomen and head of O. formosanus. The knockdown of IDH resulted in metabolic disorders in the whole organism. The dsIDH-injected workers showed significantly reduced walking activity but increased foraging success. Interestingly, IDH knockdown altered brain energy metabolism, resulting in a decline in ATP levels and an increase in IDH activity. Additionally, the social context affected brain energy metabolism and, thus, altered foraging behavior in O. formosanus. We found that the presence of predator ants increased the negative influence on the foraging behavior of dsIDH-injected workers, including a decrease in foraging success. However, an increase in the number of nestmate soldiers could provide social buffering to relieve the adverse effect of predator ants on worker foraging behavior. Our orthogonal experiments further verified that the role of the IDH gene as an inherent factor was dominant in manipulating termite foraging behavior compared with external social contexts, suggesting that energy metabolism, especially brain energy metabolism, plays a crucial role in regulating termite foraging behavior.
All behaviors consume energy (to various degrees), and some also facilitate energy acquisition, such as foraging behavior, which presents a cost–benefit trade-off between growth and survival in organisms (Mathot and Dingemanse 2015; Sandhu et al. 2018). The foraging behavior of social insects is an extremely complex process involving the self-organization of a large number of individuals to collect foods from various sources (Camazine et al. 2001; Sumpter and Pratt 2003). For example, the honey bee Apis mellifera recruits additional foragers to valuable resources by dancing to signal the location of the resources to their nestmates (Barron and Plath 2017). In seed-harvesting ants of Pogonomyrmex spp., a mixture of individual and group foraging is the most common foraging strategy (Warburg et al. 2017). A group of foraging workers utilizes clay to build structural supports to increase foraging resources in the termite Coptotermes acinaciformis (Oberst et al. 2016). Although foraging insects usually need a high energy cost during their long foraging trips, they can always achieve colony’s energetic optimization through appropriate behavioral strategies (Mathot and Dingemanse 2015). For instance, Eciton army ants could construct complex bridges from their own bodies to span gaps in the colony’s foraging trail, representing a cost–benefit trade-off (Garnier et al. 2013; Reid et al. 2015). In foraging honeybees, they minimized costs of foraging and optimized energetic efficiency via flexible change of strategies, such as “economizing” strategy or “investment-guided” strategy (Stabentheiner and Kovac 2014). Therefore, social insects always prefer to employ highly effective and energy-saving foraging strategies to obtain sufficient food and thereby fulfill the energy requirements of their colonies.
A large body of research is focused on the relationship between energy metabolism and behavior in both vertebrates and invertebrates (Yeates et al. 2007; Plaçais and Preat 2013; Haagensen et al. 2014; Mathot and Dingemanse 2015), revealing that the brain is a major regulator of behavior (Feinerman and Traniello 2016; Rittschof and Schirmeier 2018). For instance, in adult Drosophila, the knockdown of glycolytic enzymes specifically in the glial cells of the nervous system in the brain leads to severe locomotor deficits (Volkenhoff et al. 2015). In addition, neural regulation of behavior requires large amounts of energy in the brain of the cricket Gryllus campestris L. (Huber 1978). It seems that the basic energy metabolism related to neuromodulation can explain the relationship between energy metabolism and behavior (Rittschof et al. 2015, 2018). Isocitrate dehydrogenase (IDH) is an important rate-limiting enzyme that catalyzes the decarboxylation of isocitrate to alpha-ketoglutarate in the tricarboxylic acid cycle (TCA) and generates nicotinamide-adenine dinucleotide (NADH), which feeds into oxidative phosphorylation to generate adenosine triphosphate (ATP) (Nation 2015). The environmental conditions could change the expression level of IDH. For example, there was significant correlation between idh-1 allele frequencies and environmental conditions (temperature and rainfall) in the cricket Allonemobius socius (Huestis and Marshall 2006; Huestis et al. 2009). The increased expression of IDH enzyme had been found in S form female malaria mosquitoes Anopheles gambiae under dry conditions (Hidalgo et al. 2014). Although the role of metabolic enzymes in the behavioral neuromodulation has been verified, how the expression of metabolic genes impact foraging behavior in social insects under different environmental conditions is still unknown.
Actually, complex social contexts can affect foraging behavior in social insects. Considerable research has shown that predation risk seriously affects animal activity and foraging behavior (Lima 1988; Nonacs and Dill 1990; Krams 2000; Fraser et al. 2004; Vijayan et al. 2019). Individuals may make collective decisions in intricate social contexts (Frank and Eduard 2017) and then respond to the presence of predators by showing a range of behavioral and physiological changes to reduce the impact of increased energy expenditure (Tian et al. 2017). For instance, cryptic termites can change their foraging strategy by eavesdropping on vibrational cues from the footsteps of predatory ants to limit predation risk (Hughes et al. 2012; Oberst et al. 2017). Honey bees avoid flowers with crab spiders and flowers that have recently held spiders during foraging (Readera et al. 2006). Therefore, predation pressure is an important factor impacting foraging behavior in social insects. In addition, social information provided by nestmates can also change individual foraging decisions (Josens et al. 2016). In social insects, soldiers play a vital role in coping with the risk of external predation by sending out warning signals in a special way so that the other nestmates can avoid risks in a limited time through nestmate recognition during the process of foraging (Tian and Zhou 2014; Lucas et al. 2018; Sun et al. 2019). Social buffering is involved in the ability of neighboring individuals in a colony to reduce the negative impact of stressors on other individuals in some social insects (Scharf et al. 2012; Tian et al. 2017). For example, in the termite Reticulitermes flavipes, the presence of nestmate soldiers can decrease energetically costly actions of workers, and then change the ability of workers to cope with the competition risk imposed by conspecific non-nestmates (Tian et al. 2017). However, it is unclear what behavioral changes take place in foraging termites that have different energy levels and that are subjected to the presence of predator ants and to social buffering through nestmate soldiers.
The subterranean termite Odontotermes formosanus (Shiraki), which is a fungus-cultivating higher termite, can construct large underground cavities and damage many kinds of trees (Wen et al. 2014; Chiu et al. 2018). Workers exhibit large foraging areas and long foraging distances in the termite O. formosanus (Huang et al. 2006). They need to build shelter tubes during their foraging to avoid adverse environmental factors and increase foraging resources (Cornelius and Osbrink 2010; Huang et al. 2012; Xu et al. 2019). Thus, sufficient energy reserves may be an important factor affecting the likelihood of foraging success in termites (Briffa and Elwood 2005). In this study, we hypothesized that IDH knockdown might impair energy metabolism and alter the performance of foraging behavior in termites under different social contexts (with or without predator ants or/and nestmate soldiers). Thus, we cloned the IDH gene encoding the α subunit of IDH and observed its expression pattern in O. formosanus. Subsequently, we silenced the IDH gene and explored the effect of energy metabolism alterations in the whole organism and brain on the foraging behavior of O. formosanus. Moreover, we carried out orthogonal experiments to analyze the changes in IDH-mediated foraging behavior in different social contexts (with or without Leptogenys kitteli predator ants or/and nestmate soldiers) according to 4 experimental parameters (velocity, distance moved, frequency, and cumulative time in food zones) in O. formosanus.
Materials and Methods
Experimental termites
We collected termite samples from 27 O. formosanus colonies in the field (Supplementary Table S1). Samples of ants were collected as predators from a single L. kitteli colony in the field. All the termite and ant colonies were collected from Shizi Hill, Wuhan City, China. They were raised under controlled laboratory conditions (darkness, 25 ± 1°C, 80 ± 5% relative humidity). Healthy termite and ant samples were chosen for the subsequent experiments.
Cloning and sequencing of the IDH gene
The termite samples were frozen in liquid nitrogen immediately after collection. RNA was extracted with RNAiso Plus (Takara Bio Inc., Tokyo, Japan), and cDNA was synthesized using a PrimeScript RT reagent kit with gDNA Eraser (Takara Bio Inc.). Gene-specific primers for the complete open-reading frame (ORF) of IDH were designed based on partial sequences of Unigene 32825 obtained from the transcriptome data of the heads of O. formosanus workers (Huang et al. 2012). The nested polymerase chain reaction (PCR) amplification reactions were carried out as follows: 95°C for 4 min; 38 cycles of 95°C for 30 s, 55°C for 30 s, and 72°C for 80 s; and 1 cycle at 72°C for 5 min. Following PCR amplification, the IDH fragment was cloned, purified, and sequenced.
Sequence and phylogenetic analyses of the IDH gene
Sequence alignment and analysis were performed using the BLAST service of the National Center for Biotechnology Information (NCBI, http://www.ncbi.nlm.nih.gov). Protein multiple alignment analyses were performed using MEGA 6.0 and GeneDoc 2.0 software. The deduced amino acid sequence of IDH was aligned with its corresponding orthologs from the harvester ant Pogonomyrmex barbatus (XP_011645897.1), the honey bee A. mellifera (XP_006564183.2), and Homo sapiens (NP_005521.1). cDNA and amino acid sequence similarity searches were performed using the BLAST algorithm (https://blast.ncbi.nlm.nih.gov/Blast.cgi). The phylogenetic relationships of IDH and the homologous genes in other species were analyzed using the neighbor-joining (NJ) method with MEGA 6.0 software with 1,000 bootstrapping replicates. The prediction of protein secondary structure was performed using the Self-Optimized Prediction Method with Alignment algorithm of the online software PRABI-Lyon-Gerland (https://prabi.ibcp.fr/htm/site/web/home) on the NPS@ server SOPMA (Combet et al. 2000).
IDH transcription in different tissues
The head, abdomen, and thorax tissues were dissected from O. formosanus workers on dry ice. Thirty individual thorax specimens and 15 individual head and abdomen specimens were prepared for each replicate and 6 replicates were performed for the expression patterns of IDH. RNA extraction and cDNA synthesis from these body regions were carried out as described in the section on the cloning and sequencing of the IDH gene. Quantitative real-time PCR (qRT-PCR) was performed with Hieff™ qPCR SYBR® Green Master Mix (Yeasen, China) in a QuantStudio 6&7 Flex Real Time PCR System (Applied Biosystems, Life Technologies, Milan, Italy). Gene-specific primers were designed by using NCBI primer-BLAST and are presented in Supplementary Table S2. The mRNA levels were normalized to β-actin and glyceraldehyde-3-phosphate dehydrogenase (GAPDH) as the reference genes. The relative expression levels of IDH among the 3 body regions were calculated using the 2−ΔΔCt method (Livak and Schmittgen, 2001). Six biological replicates were performed for the RT-qPCR analysis of IDH. The primers used for RT-qPCR are listed in Supplementary Table S2.
RNA interference with IDH transcription
The template cDNAs were amplified by using PCR primers that had T7 RNA polymerase sequences appended to their 5′-ends. The PCR primers for the dsRNA template (shown 5′–3′) are shown in Supplementary Table S2. Injection was performed using a sterilized microinjector (SYS-PV820, World Precision Instruments, USA). Approximately 3 μg of dsRNA targeting IDH or GFP (dsIDH or dsGFP) that dissolved in 150 nL of water, or 150 nL of water was injected into the side of the thorax (Zhou et al. 2006). We used dsGFP-injected and water-injected termites as the control or reference. Water control was only conducted in the orthogonal experiments. After injection, the termites were transferred to Petri dishes with filter paper containing a 7.5% glucose solution for 1 day, 3 days, or 4 days to check the efficiency of RNA interference (RNAi), respectively. Individuals and brain tissues were collected separately at third day after injection for subsequent experiments. qPCR was performed for each sample using a QuantStudio 6&7 Flex Real-Time PCR System. Then, cDNA to be used as the template for qRT-PCR was synthesized from the total RNA of each of 6 individuals at third day after injection. Gene-specific primers were designed by using NCBI primer-BLAST and are presented in Supplementary Table S2. mRNA levels were quantified using β-actin and GAPDH as the reference genes. The relative expression levels of specific genes were calculated via the 2−ΔΔCt method (Livak and Schmittgen, 2001). Each treatment consisted of at least 6 biological replicates.
Brain tissue anatomy and immunocytochemistry with synapsin
Termite brains were dissected out in an ice-cold sterilized 0.9% sodium chloride solution in a dissecting dish cooled on ice (Ishikawa et al. 2016) and then immediately frozen with liquid nitrogen for IDH expression and metabolite assays. The insects were anesthetized by cooling on ice. The brains were dissected out and fixed in a 4% paraformaldehyde solution in phosphate-buffered saline (PBS; in mM, 684 NaCl, 13 KCl, 50.7 Na2HPO4, 5 KH2PO4, pH 7.4) for 2 h at room temperature. After fixation, the brains were rinsed in PBS for 4 × 15 min. To minimize nonspecific staining, the brains were preincubated in 5% normal goat serum (Sigma, St. Louis, MO, USA) in PBS containing 0.5% Triton X-100 (PBSX; 0.1 M, pH 7.4) for 3 h at room temperature. Then, the brains were incubated in the primary antibody SYNORF1 at 1:100 in PBSX at 4°C for 3 days. After incubation, the brains were rinsed in PBS for 4 × 15 min before being incubated in the Cy2-conjugated anti-mouse secondary antibody (dilution 1:300 in PBSX; Invitrogen, Eugene, OR), at 4°C for 1 day. The brains were finally rinsed for 4 × 15 min in PBS, dehydrated in an ascending ethanol series, and mounted in methyl salicylate (Zhao et al. 2016). A confocal laser scanning microscope (LSM 510, META Zeiss, Jena, Germany) was used to scan the brain and to obtain serial optical images with excitation by a 488 nm laser.
Metabolite assays
Three days post-injection, termite individuals were weighed and then immediately crushed in liquid nitrogen and dissolved at a ratio of 10 mg of body fresh weight to 100 μL of the solution recommended by the manufacturer. Termite brains were dissected on ice immediately after foraging assays in different social contexts including predator ants (−) + nestmate soldiers (−), predator ants (+) + nestmate soldiers (−), and predator ants (+) + nestmate soldiers (+) for 15 min (“−” in parentheses means “not present” and “+” in parentheses means “present”), and then crushed in liquid nitrogen and dissolved in the solution recommended by the manufacturer. The determination of the ATP (N = 9 replicates), NADH (N = 6 replicates), IDH (N = 6 replicates), and glucose (N = 9 replicates) contents of the whole organism and the ATP (N = 6 replicates) and IDH (N = 6 replicates) contents of the brain was performed according to the protocols provided by the manufacturers (Beyotime Biotechnology [Shanghai, China], Nanjing Jiancheng Bioengineering Institute [Jiangsu, China], and Zeye Biological Technology [Shanghai, China]). The protein concentration of the brain was determined according to the protocol of the bicinchoninic acid (BCA) protein concentration determination kit from Beijing Dingguo Changsheng Biotechnology (Beijing, China).
Termite behavioral apparatus
The circular behavioral apparatus included 1 inner ring (D = 300 mm) as the foraging area in which 10 termites tested and a 10 mm outer ring containing the predator ant L. kitteli. Both rings of the testing arena (D = 310 mm) had a 10 mm-tall wall edge. The wall of the inner ring included 40 evenly spaced 1 mm slits that allowed the transmission of chemical cues and antennal contacts but were too narrow for lethal interactions (Tian et al. 2017). Four pieces of 20 mm circular filter paper moistened with 7.5% glucose solution were placed on marked food patches as food zones, which were positioned on the different 4 directions (0°, 90°, 180°, and 270° clockwise) of the inner ring (Supplementary Figure S1). The distance between food zone and edge of inner ring was about 4 mm.
Foraging assays
Four important phenotypic parameters of foraging behavior were measured using a digital camera (BASLER, acA1920-40gc) coupled to video tracking software (EthoVision XT 14.0, Noldus Information Technology), capturing video at 25 frames per second (Gao et al. 2020), including the velocity and distance moved, and the frequency and cumulative time in food zones during foraging. In this study, the velocity and distance moved were used to describe the walking activity of the worker termites, and the frequency and cumulative time in food zones were used to describe the foraging success of the worker termites (Anreiter et al. 2017; Hughson et al. 2018).
In the foraging assays performed without regard to the social context (L. kitteli predator ants and nestmate soldiers), 10 workers injected with dsIDH or dsGFP for 3 days were placed in different 35 mm Petri dishes before the start of the experiment. The foraging assay began after the 10 workers were added into the center of the inner ring of the testing arena with moistened filter paper, and the foraging behavior of the 10 workers was then recorded by using the video tracking software EthoVision XT. After recording for 15 min, the tested workers were removed and then quickly placed in liquid nitrogen. Considering the adaptation period of termites in the behavioral apparatus, the features of the foraging behavior of the 10 workers were analyzed after 5 min of recording.
To further explore the impact of IDH on the foraging behavior of O. formosanus in different social contexts (Treatment 1–Treatment 9), we set up 9 treatment groups with or without predator ants or/and nestmate soldiers using orthogonal experimental design L9 (33) (Supplementary Table S3). In the foraging assays performed in different social contexts (with or without L. kitteli predator ants or/and nestmate soldiers), workers and soldiers who did not receive any injection were individually marked with red color on the abdomen by using PX-21 uni-Paint markers prior to the assay so that each individual could be identified. To reduce the potential for injury, each body part of an individual was only marked once (Tian et al. 2017). For the convenience of experimental operation, different injected termites were placed in different 35 mm Petri dishes before the start of the experiment. For example, the 24 dsIDH-injected termites were transferred to 3 35 mm Petri dishes, and each Petri dish contained 8 termites. Similarly, the 24 termites that were injected with dsGFP or water were transferred to Petri dishes, respectively. Each Petri dish was covered with filter paper moistened with water. According to orthogonal experimental design L9 (33) (Supplementary Table S3), we transferred 1 or 2 marked workers or/and marked soldiers that were not subjected to dsRNA or water injection separately into 9 Petri dishes. Finally, there were a total of 10 termites in each Petri dish, including unmarked and marked individuals.
At the beginning of the experiments, the inner ring of the testing arena was covered with moistened filter paper. According to the orthogonal experimental design L9 (33) (Supplementary Table S3), we placed different numbers of L. kitteli predator ant workers in the outer ring of the testing arena for 30 min before adding termites. The foraging assay began after the 10 termites (including workers and/or soldiers) were added to the center of the inner ring of the testing arena with moistened filter paper. The features of the foraging behavior of the 10 termites were recorded by using EthoVision XT software. After recording for 15 min, the tested termites were removed and then quickly placed in liquid nitrogen. The features of the foraging behavior of 8 workers were analyzed after 5 min of recording, which was also the procedure for the foraging assay without the social context described above.
Statistical analysis
All statistical analyses were conducted using IBM SPSS Statistics 19.0 software. The Shapiro–Wilk test was used to verify whether the data conformed to a normal distribution or not. The expression pattern of IDH and the phenotypic parameters of foraging behavior in the orthogonal experiments showed a normal distribution and were analyzed with Tukey’s HSD test. RNAi efficiency in the whole organism showed a normal distribution and were analyzed with Paired t-test. The phenotypic parameters of foraging behavior after silencing IDH without regard to the social context showed a normal distribution and were analyzed with Independent t-test. The impact degrees of 3 factors (IDH, predator number, and soldier number) on worker foraging behavior were determined with a generalized linear model (GLM). The assessment of RNAi efficiency in the brain and metabolite assays showed no normal distribution and was performed with the Wilcoxon rank-sum test. The significance level in this study was set at α = 0.05.
Results
Cloning, expression, and RNAi knockdown efficiency of the IDH gene
The complete ORF of IDH was 1,074 bp and encoded a predicted protein of 357 amino acids (aa), which is highly conserved in social organisms (Figure 1A). The prediction of the secondary structure of the IDH protein demonstrated that the amino acid sequence contained 47.90% alpha-helices, 10.36% extended strands, 6.16% beta-turns, and 35.57% random coils (Figure 1B). The analysis of the conserved domains with the NCBI tool indicated that IDH presented the specific hit Iso_dh (26–350 aa) (Figure 1B). The evolutionary tree (Figure 1C) showed that IDH from O. formosanus was clustered with that from the German cockroach, Blattella germanica, and 2 termite species, Zootermopsis nevadensis and Cryptotermes secundus.
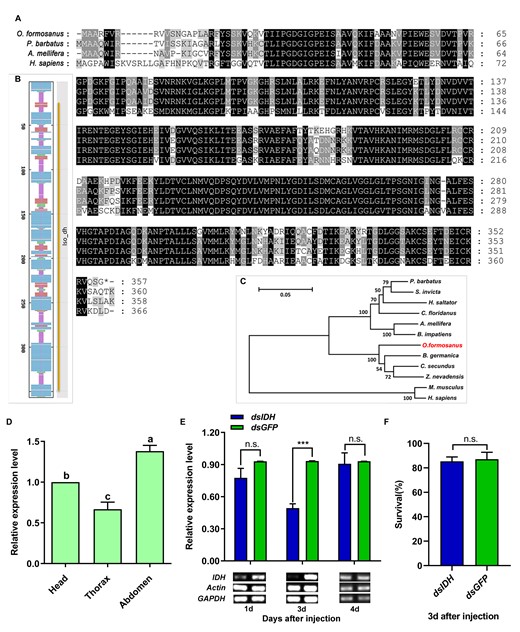
Cloning, expression, and RNAi efficiency of the IDH gene in O. formosanus. (A) Multiple alignments of the amino acid sequences deduced for IDH in O. formosanus and 3 other social species. Identical residues were shaded in black. Dashed lines indicated the gaps. (B) Secondary structure prediction of the amino acid sequence of IDH and conserved domains of IDH. Random coils were shown in purple, alpha-helixes were shown in blue, beta-turns were shown in green, and extended strands were shown in red. The gene showed an Iso_dh-specific hit (26-350). (C) Phylogenetic relationships of the IDH proteins from O. formosanus and 11 other species. (D) Expression patterns of IDH in the head, thorax, and abdomen of workers (N = 6 samples/treatment, 15 heads/sample, 15 abdomens/sample, 30 thoraxes/sample). (E) Expression level and semiquantitative detection of IDH 1, 3, and 4 days after dsRNA injection in workers (N = 9 samples/treatment, 5 termites/sample). (F) Survival 3 days after dsRNA injection in workers (N = 6 samples/treatment, 5 termites/sample). The data are presented as mean ± SEM. Different lowercase letters in a column indicate significant differences by Tukey’s HSD test. ***P < 0.001, n.s. means no significant difference.
Our results showed that IDH expression in the abdomen and head was significantly higher than that in the thorax (Figure 1D, Tukey’s HSD test, F = 30.380, df = 2, 15, P < 0.001, N = 6). The expression level of IDH was significantly decreased by 47.44% 3 days after the introduction of RNAi (Figure 1E, Paired t-test, t = −11.055, df = 8, P < 0.001, N = 9). Semiquantitative RT-PCR analysis also showed that the expression of IDH was successfully inhibited (Figure 1E). In addition, there were no significant differences in survival between dsIDH-injected individuals and dsGFP-injected individuals (Figure 1F, Paired t-test, t = −0.419, df = 5, P = 0.692, N = 6).
IDH knockdown reduced the energy supply but increased glucose accumulation
The IDH enzyme is an important rate-limiting enzyme in the TCA and can catalyze the decarboxylation of isocitrate to alpha-ketoglutarate (Nation 2015). Therefore, we silenced IDH to investigate the changes in the NAD+-IDH reaction in the TCA and the level of glucose 3 days after the injection of dsIDH. The results showed that IDH activity (Figure 2A, Wilcoxon rank-sum test, Z = −2.023, P = 0.043, N = 6), ATP levels (Figure 2B, Wilcoxon rank-sum test, Z = −2.666, P = 0.008, N = 9), and NADH levels (Figure 2C, Wilcoxon rank-sum test, Z = −2.201, P = 0.028, N = 6) were significantly decreased in dsIDH-injected termites compared with dsGFP-injected termites. However, the glucose level was significantly increased compared with that in dsGFP-injected termites (Figure 2D, Wilcoxon rank-sum test, Z = −2.192, P = 0.028, N = 9).
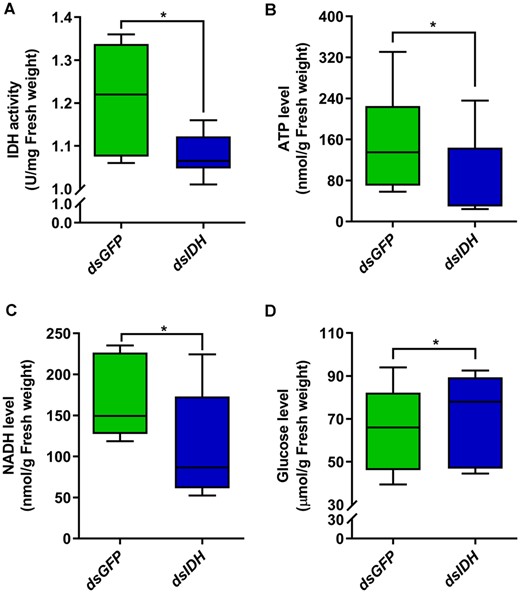
Alterations in energy metabolism after silencing IDH in O. formosanus. (A) IDH activity, (B) ATP levels, (C) NADH levels, and (D) glucose levels were detected by different test kits 3 days after dsIDH injection (N = 6 samples/treatment, 5 termites/sample). The data are presented as mean ± SEM. Wilcoxon rank-sum test, *P < 0.05.
IDH knockdown decreased walking activity but increased foraging success
To investigate the relationship between energy metabolism and foraging behavior, we silenced IDH by injecting dsIDH and then observed the phenotypic changes in the foraging behavior (Figure 3A) of O. formosanus. We found that the foraging trajectories of dsGFP-injected individuals were denser than those of dsIDH-injected individuals (Figure 3B). Both the distance moved (Figure 3C, Independent t-test, t = −2.116, df = 18, P = 0.049, N = 10) and velocity (Figure 3D, Independent t-test, t = −2.140, df = 18, P = 0.046, N = 10) were significantly decreased in dsIDH-injected individuals compared with dsGFP-injected individuals. However, the frequency in food zones (Figure 3E, Independent t-test, t = 2.191, df = 18, P = 0.042, N = 10) was significantly increased and the cumulative time in food zones (Figure 3F, Independent t-test, t = 2.076, df = 18, P = 0.053, N = 10) was marginally significantly increased in dsIDH-injected individuals compared with dsGFP-injected individuals.
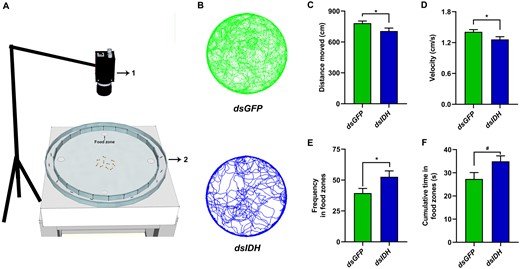
Silencing IDH decreased walking activity but increased foraging success in O. formosanus. (A) Behavioral apparatus (1, camera; 2, testing arena). Four pieces of food patches as food zones were positioned on the different 4 directions (0°, 90°, 180°, and 270° clockwise) of the inner ring. (B) Foraging trajectories of dsIDH-injected (deep green color) and dsGFP-injected (deep blue color) workers. (C) Distance moved, (D) velocity, (E) requency in food zones, and (F) cumulative time in food zones of dsIDH-injected and dsGFP-injected workers (N = 10 samples/treatment, 10 termites/sample). The data are presented as mean ± SEM. Independent t-test, *P < 0.05. #0.05 ≤ P < 0.1.
Effect of IDH on brain energy metabolism in different social contexts
We successfully obtained and observed the brain tissue of worker termites through the immunocytochemistry analysis of synapsin (Figure 4A,B). Our results showed that IDH expression in the worker brain was significantly decreased 3 days after dsIDH injection (Figure 4C, Wilcoxon rank-sum test, Z = −2.201, P = 0.028, N = 6). In 3 different social contexts, IDH activity in the brains of dsIDH-injected worker individuals was significantly higher than that in dsGFP-injected worker individuals, respectively (Figure 4D, Wilcoxon rank-sum test, Z1 = −1.992, P1 = 0.046, n1 = 6; Z2 = −2.201, P2 = 0.028, n2 = 6; Z3 = −2.201, P3 = 0.028, n3 = 6), while ATP levels in the brains of dsIDH-injected worker individuals were significantly lower than that in dsGFP-injected worker individuals, respectively (Figure 4E, Wilcoxon rank-sum test, Z1 = −2.201, P1 = 0.028, N1 = 6; Z2 = −2.201, P2 = 0.028, N2 = 6; Z3 = −2.201, P3 = 0.028, N3 = 6). Additionally, we found that IDH activity in the worker brain was significantly higher in the social context with predator ants and soldiers than that in the social context without predator ants and soldiers (Figure 4D, Wilcoxon rank-sum test, dsIDH: Z = −1.992, P = 0.046, N = 6; dsGFP: Z = −2.201, P = 0.028, N = 6). However, ATP levels in the worker brain were significantly lower in the social context with predator ants and soldiers than that in the social context without predator ants and soldiers (Figure 4E, Wilcoxon rank-sum test, dsIDH: Z = −2.201, P = 0.028, N = 6; dsGFP: Z = −2.201, P = 0.028, N = 6).
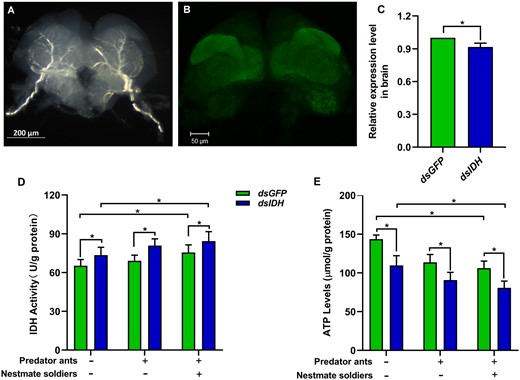
Effect of IDH silencing on brain energy metabolism in O. formosanus in different social contexts. (A) Full brain tissue of workers. (B) Confocal image of the worker brain. (C) Expression level of IDH in the brains of workers 3 days after dsIDH injection (N = 6 samples/treatment, 20 brains/sample). (D) IDH activity and (E) ATP levels in the brains of dsIDH-injected and dsGFP-injected workers in different social contexts (N = 6 samples/treatment, 20 brains/sample). The data are presented as mean ± SEM. Wilcoxon rank-sum test, *P < 0.05.
Influence of IDH on foraging behavior in different social contexts
Our results showed that the foraging trajectories of O. formosanus in the 9 treatment groups varied, which was associated with the status of worker energy metabolism and different social contexts (Figure 5E). The foraging trajectories of water-injected workers became increasingly chaotic instead of walking along edges when along with the increase in the number of predators (from Treatments 1 to 3 with all water treatments). The foraging trajectories of dsGFP-injected workers were similar to those of water-injected workers (from Treatments 4 to 6 with all dsGFP treatments). However, the foraging trajectories of dsIDH-injected workers exhibited simply and lower flexibility when the number of predators increased (from Treatments 7 to 9 with all dsIDH treatments).
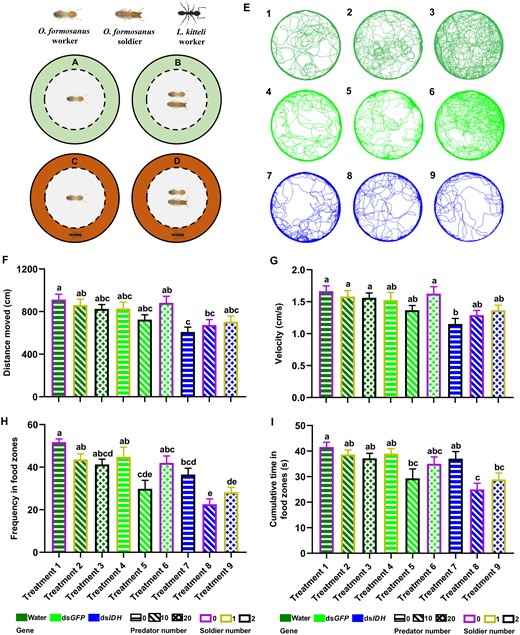
The changes of foraging behavior after IDH silencing in O. formosanus in different social contexts. Workers without (A) or with (B) soldiers were confined in the inner ring (in gray) without predator ants in the outer ring (areas in light green). The wall of the center dish was cut vertically to make 1 mm-wide silts. Predation risk was perceived by the antennation of workers of the ant L. kitteli through the slits. The predator ants were placed in the outer ring (areas in deep orange) without (C) or with (D) soldiers. (E) Representative maps of foraging trajectories in the water-injected (in deep green), dsGFP-injected (in green), and dsIDH-injected (in blue) workers (N = 14 samples/treatment, 8 termites/sample). Numbers in the upper left corner of each trajectory map indicate different treatment numbers in the orthogonal experiments L9 (33) (Treatment 1: water + 0 predator ant + 0 nestmate soldier; Treatment 2: water + 10 predator ants + 1 nestmate soldier; Treatment 3: water + 20 predator ants + 2 nestmate soldiers; Treatment 4: dsGFP + 0 predator ant + 1 nestmate soldier; Treatment 5: dsGFP + 10 predator ants + 2 nestmate soldiers; Treatment 6: dsGFP + 20 predator ants + 0 nestmate soldier; Treatment 7: dsIDH + 0 predator ant + 2 nestmate soldiers; Treatment 8: dsIDH + 10 predator ants + 0 nestmate soldier; Treatment 9: dsIDH + 20 predator ants + 1 nestmate soldier). (F) Distance moved, (G) velocity, (H) frequency in food zones, and (I) cumulative time in food zones of 9 treatments (N = 14 samples/treatment, 8 termites/sample). The data are presented as mean ± SEM, and different lowercase letters in a column indicate significant differences between groups by Tukey’s HSD test, P < 0.05.
There were significant differences in the phenotypic features of foraging behavior among the 9 treatment groups in worker termites. The distance moved in Treatment 7 was significantly shorter than that in Treatments 1, 2, and 6 (Figure 5F, Tukey’s HSD test, F = 3.994, df = 8, 117, P < 0.001). The distance moved in Treatment 8 was significantly shorter than that in Treatment 1 (Figure 5F, Tukey’s HSD test, F = 3.994, df = 8, 117, P = 0.042). At the same time, the velocity in Treatment 7 was much lower than that in Treatments 1, 2, 3, and 6 (Figure 5G, Tukey’s HSD test, F = 3.648, df = 8, 117, P = 0.001). However, the frequency in food zones in Treatment 5 was significantly less than that in Treatments 1, 2, and 4, and the frequency in food zones in Treatment 8 was significantly lower than that in Treatments 1, 2, 3, 4, 6, and 7 (Figure 5H, Tukey’s HSD test, F = 9.272, df = 8, 117, P < 0.001). Additionally, the cumulative time of visits in food zones in Treatment 8 was significantly shorter than that in Treatments 1, 2, 3, 4, and 7 (Figure 5I, Tukey’s HSD test, F = 4.981, df = 8, 117, P < 0.001). The cumulative time of visits in food zones in Treatment 9 was also significantly shorter than that in Treatment 1 (Figure 5I, Tukey’s HSD test, F = 4.981, df = 8, 117, P = 0.014).
The impact degrees of 3 factors on the velocity and distance moved were as follows: IDH expression > soldier number > predator number, but the impact degrees of the 3 factors on the frequency and cumulative time in food zones were as follows: IDH expression > predator number > soldier number. Among these factors, IDH expression showed a significant influence on the velocity and distance moved, and frequency and cumulative time in food zones (Supplementary Table S4, GLM, all P < 0.001). Additionally, soldier number exhibited a significant influence on the distance moved and a marginally significant influence on the velocity (Supplementary Table S4, GLM, P1 = 0.044; P2 = 0.060). Predator number exerted a significant influence on the frequency and cumulative time in food zones (Supplementary Table S4, GLM, P1 < 0.001; P2 < 0.001).
Discussion
Foraging behavior requires integration across the different body regions (Matsumura and Miyatake 2018). In this study, we found that the expression levels of IDH in the abdomen and head were higher than thorax (Figure 1D), indicating that different body regions have different investment of energy (Lease and Wolf 2011). Additionally, the silencing IDH disrupted mitochondrial metabolism, including the disruption of the NAD+-IDH reaction in the TCA cycle and the reduction of ATP levels, NADH levels, and IDH activity (Figure 2A–C), which caused decreased walking activity, including shorter collective distances moved and slower velocities. The starvation could lead to the same physiological consequences as IDH-knockdown did. For instance, the activity of IDH enzymes was also relatively low in prolonged starved carp Cyprinus carpio and tilapia Oreochromis niloticus (Shimeno et al. 1996). Additionally, the trajectories of worker termites showed lower mobility (Figure 3B). These results suggested that the impaired metabolism might damage neuronal and muscle function and thus decreased the output locomotion of termites, which was consistent with the behavioral changes in the adult Drosophila with knockdown of glycolytic enzymes (Volkenhoff et al. 2015). Normally, glucose can be decomposed and then produce ATP through the glycolysis pathway and TCA cycle (Nation 2015). However, a significant increase in glucose content was found in this study after silencing IDH. The similar results were also found in the downregulation of human IDH3α (Zhang et al. 2015) and the knockdown of the termite R. chinensis IDH (Liu et al. 2020). Our results indicated that silencing IDH might disrupt the process of glucose metabolism and resulted in the accumulation of glucose. Surprisingly, knocking down IDH increased the frequency and cumulative time in food zones within a certain amount of foraging time, suggesting that termite colonies could ensure the food supply by increasing foraging success (Anreiter et al. 2017) and prolonging the time of food acquisition to reduce the negative effects of energy deficiency. This behavioral strategy of workers during foraging was different from walking alone, which was displayed during a non-stationary behavioral process (Bartumeus et al. 2016; Hiroyuki et al. 2020). In addition, the reason of glucose accumulation may include the increased feeding amount because the frequency and cumulative time in the food zones were increased in dsIDH-injected termites. Our results demonstrated that the changes in the energy metabolism of the whole organism caused by IDH knockdown in this study reduced the walking activity of termites at the same time increasing the foraging success.
IDH knockdown significantly reduced ATP levels but dramatically improved IDH activity in the brains of worker termites after a period of foraging in the social contexts including predator ants (−) + nestmate soldiers (−), predator ants (+) + nestmate soldiers (−), and predator ants (+) + nestmate soldiers (+). These results suggested that the production efficiency of ATP decreased. Usually, glucose is metabolized via glycolysis and the TCA cycle to generate sufficient ATP through the process of oxidative phosphorylation (Lunt and Vander Heiden 2011). However, IDH downregulation disrupts the TCA cycle and thereby reduces the generation of ATP. On the other hand, it has been demonstrated that a decrease in the expression of IDH3α converts the metabolic mode from oxidative phosphorylation to aerobic glycolysis in cancer-associated fibroblasts (Zhang et al. 2015). However, glycolysis is far less efficient than the TCA cycle coupled to oxidative phosphorylation for the production of ATP (Lunt and Vander Heiden 2011). Therefore, the decreasing ATP levels in the brain of dsIDH-injected termites suggest that the brain might choose an inefficient way of ATP production, such as aerobic glycolysis, resulting in a low efficiency of ATP generation. Actually, ATP is necessary to maintain homeostasis and cell survival, and the loss of intracellular ATP may result in cell necrosis or apoptosis (Lunt and Vander Heiden 2011; Chandrasekaran et al. 2015). In addition, energetic constraints play a major role in neural plasticity and brain health (Niven and Laughlin 2008). Therefore, during foraging process, the shortage of energy supply and the increase of energy consumption made the ATP level decrease significantly when compared with the control in dsIDH-injected termites, while the increased IDH activity in the brain could alleviate the excessive decrease of ATP level.
Some researchers have suggested that social information can change gene expression in the brain to influence behaviors in social insects (Robinson et al. 2008). We also found that social information could affect foraging behavior by changing brain metabolic activity in termites. In this study, the decrease in ATP became more significant, and the increase in IDH activity was greater when soldiers and ants were present concurrently than those without soldiers and ants. When worker termites are exposed to predation risks during foraging, they may exhibit an aggressive state and consume much energy (Tian and Zhou 2014). At this moment, ATP levels decreased and the IDH activity increased in the brains of dsIDH- and dsGFP-injected foragers in the social context with predators, suggesting that the degree of aerobic glycolysis might be enhanced, as reported for the metabolic pathway in the brain of aggressive honey bees (Chandrasekaran et al. 2015). In other words, the brains of worker termites may prefer an inefficient but faster pathway for ATP production (Lunt and Vander Heiden 2011) to meet the high energy demands of foraging behavior when they are threatened by predators. On the other hand, the addition of nestmate soldiers during foraging may enhance the group cognition that is energetically costly in brains in order to cooperatively cope with the threat from the predator ants (Feinerman and Traniello 2016). In addition, the social context may regulate the expression of genes associated with encoding neuropeptides in the brain, such as gonadotropin-releasing hormone (GnRH), vasotocin (VT), and vasopressin (VP) (White et al. 2002; Goodson et al. 2009). The sensory and higher-order integrative processing mechanisms that are used when insects behave in complex social contexts are also associated with nervous systems of the brain composed of a small number of neurons and relatively simple circuitry (Kamhi and Traniello 2013), and the energetic basis of these behaviors is a bridge between behavioral ecology and neuroscience (Rittschof et al. 2015). Moreover, intermediates of energy metabolism can impact the concentration of neurotransmitters by regulating the synthesis of precursors such as alpha-ketoglutarate, which is the precursor of the excitatory neurotransmitter glutamate produced via the TCA cycle (Li-Byarlay et al. 2014; Chandrasekaran et al. 2015). Thus, the changes in energy metabolism in the brain caused by IDH downregulation and social context might alter the energetic and nervous system bases of foraging behavior in termites.
Silencing IDH reduced the walking activity of worker termites in Treatments 7–9 (all dsIDH treatments) and simplified their foraging trajectory (Figure 5E). This reduction in walking activity of Treatment 7 decreases significantly when the number of nestmate soldiers was increased but no predators were present compared with the water- or dsGFP-injected workers in Treatments 1, 2, and 6 (water + 0 or 1 nestmate soldier and dsGFP + 0 nestmate soldier), indicating that worker termites may choose a more profitable foraging strategy, such as reducing their foraging area, to relieve the energy shortage (Hughes and Ward 1993; Thomson et al. 2012) because more energy may be needed to cover a larger movement space (Tamburello et al. 2015; Campos-Candela et al. 2019). When predator ants were present without nestmate soldiers, the foraging success in Treatment 8 (dsIDH + 10 predator ants + 0 nestmate soldier) decreased sharply compared with Treatment 7 (dsIDH + 0 predator ant + 2 nestmate soldier), indicating that predator ants seriously affect foraging behavior and that the absence of nestmate soldiers means that foraging workers face a greater risk of predation if worker termites forage for a long time and feed on a large amount of food when the energy supply is lacking (Sandhu et al. 2018). When predator ants were present, the increasing number of soldiers enhanced the frequency and cumulative time in food zones appropriately in Treatment 9 (dsIDH + 1 nestmate soldier + 20 predator ants) than Treatment 8 (dsIDH + 0 nestmate soldier + 10 predator ants), illustrating that soldiers can provide social buffering to cover the damage caused by the increase in predators during foraging (Hennessy et al. 2009; Ishikawa and Miura 2012; Tian et al. 2017).
Generally, a reduction in walking activity levels is one of the most commonly reported responses of prey to predators (Lima 1998). However, in this study, we found that the walking activity had no significant difference when predator ants increased (from Treatments 1 to 3 and Treatments 4 to 6). On the other hand, termites were more inclined to walk toward center of the arena instead of walking along edges when predators increased, suggesting that the increase in predators led to more purposeless walking, which is more energy demanding (Nonacs and Dill 1990; Vijayan et al. 2019). In addition, the walking activity and foraging success of the worker termites showed no significant changes as the number of soldiers was increased together with the number of predators. Therefore, the presence of nestmate soldiers is crucial to reduce the purposeless walking of foraging workers and relieve the predation pressure from ants to help foragers to accurately find food sites (Tian et al. 2017). Even though the increase in nestmate soldiers could decrease the purposeless walking caused by a small number of predators, foraging success still decreased significantly when the number of soldiers increased in Treatment 5 (2 nestmate soldiers + dsGFP) compared with Treatment 1 (0 nestmate soldier + water). In addition, the results of main effect analysis in the orthogonal experiments showed that IDH expression extremely significantly impacted both walking activity and foraging success in foraging workers, but nestmate soldiers only significantly influenced walking activity and predator ants only significantly influenced foraging success in foraging workers, strongly suggesting that the inherent gene plays the dominant role in modulating foraging behavior in termites compared with the external social context.
In summary, the present study verified that the metabolic gene IDH was an important regulator of foraging behavior in termites, which can be influenced by different social contexts (Figure 6). However, whether the change in brain energy affects neural functions that may be related to foraging behavior needs to be further resolved. For the subterranean termite O. formosanus, our open arena assays may have the limitation in studying their foraging behavior since we did not use the soil. However, the setup of the open arena is convenient for the observation and quantification of behavioral parameters in termites. Our findings enrich the understanding of energetic basis and strategy of termite foraging behavior in different social contexts, which will be beneficial for clarifying the role of energy supply and consumption in determining behavioral evolution in social insects.
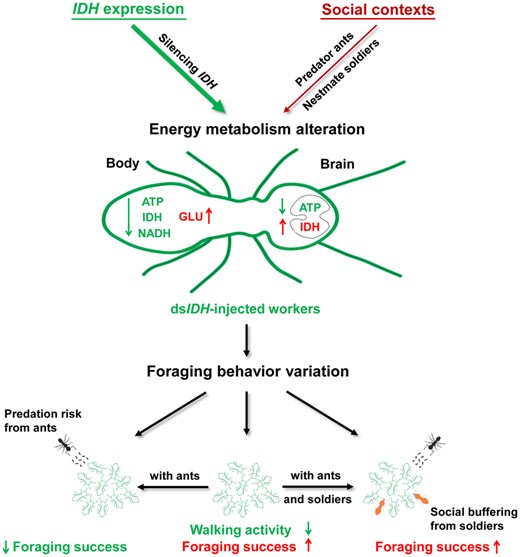
The role of the IDH gene and the social context in regulating the foraging behavior of O. formosanus. IDH silencing impaired the NAD+-IDH reaction in the TCA, leading to a decrease in ATP levels, IDH activity, and the NADH levels but an increase in glucose levels in the whole organism, resulting in increased IDH activity but decreased the ATP level in the brain. When dsIDH-injected workers foraged together, their velocity and distance moved decreased, but their frequency and cumulative time in food zones increased, suggesting that IDH downregulation reduced walking activity but enhanced foraging success. The social context could also alter the brain energy metabolism of foraging workers, including decreasing ATP levels but the increasing IDH activity in the social context with predators and soldiers, which further changed the foraging behavior of the workers. When predator ants were present, the dsIDH-injected workers decreased their frequency and cumulative time in food zones, showing a significant decline in foraging success. However, the increase in the number of nestmate soldiers strengthened social buffering to relieve the negative effect of predator ants on worker foraging behavior and, thus, improved the foraging success of dsIDH-injected workers. Our orthogonal experiments verified that the role of the IDH gene as an inherent factor was dominant in modulating termite foraging behavior compared with the external social context (predator ants and nestmate soldiers). Thus, abnormal energy metabolism mediated by IDH altered termite foraging behavior in different social contexts.
Author Contributions
H.X. and Q.H.: conceiving of and formulating the project. H.X.: performing the experimental work and writing the manuscript. H.X., Q.H., Y.G., and J.W.: analysis of data. Q.H., Y.G., J.W., A.H., and Y.L.: editing the manuscript. Q.H.: funding and overall project design.
Acknowledgments
The authors would like to thank Dr. Zhao Xincheng from Henan Agriculture University for technical help with the brain tissue anatomy and immunocytochemistry of termites. They also thank Dr. Zhang Shichang from Hubei University for the edit of this article.
Funding
This work was supported by the Fundamental Research Funds for the National Natural Science Foundation of China [grant number 31772516].
Conflict of Interest
The authors declare no competing financial interests.
Supplementary Material
Supplementary material can be found at https://dbpia.nl.go.kr/cz.