-
PDF
- Split View
-
Views
-
Cite
Cite
Stiven Roytman, Rebecca Paalanen, Alexis Griggs, Simon David, Chatkaew Pongmala, Robert A Koeppe, Peter J H Scott, Uros Marusic, Prabesh Kanel, Nicolaas I Bohnen, Cholinergic system correlates of postural control changes in Parkinson’s disease freezers, Brain, Volume 146, Issue 8, August 2023, Pages 3243–3257, https://doi.org/10.1093/brain/awad134
- Share Icon Share
Abstract
Postural instability and freezing of gait are the most debilitating dopamine-refractory motor impairments in advanced stages of Parkinson’s disease because of increased risk of falls and poorer quality of life. Recent findings suggest an inability to efficaciously utilize vestibular information during static posturography among people with Parkinson’s disease who exhibit freezing of gait, with associated changes in cholinergic system integrity as assessed by vesicular acetylcholine transporter PET. There is a lack of adequate understanding of how postural control varies as a function of available sensory information in patients with Parkinson’s disease with freezing of gait. The goal of this cross-sectional study was to examine cerebral cholinergic system changes that associate with inter-sensory postural control processing features as assessed by dynamic computerized posturography and acetylcholinesterase PET.
Seventy-five participants with Parkinson’s disease, 16 of whom exhibited freezing of gait, underwent computerized posturography on the NeuroCom© Equitest sensory organization test platform, striatal dopamine, and acetylcholinesterase PET scanning. Findings demonstrated that patients with Parkinson’s disease with freezing of gait have greater difficulty maintaining balance in the absence of reliable proprioceptive cues as compared to those without freezing of gait [β = 0.28 (0.021, 0.54), P = 0.034], an effect that was independent of disease severity [β = 0.16 (0.062, 0.26), P < 0.01] and age [β = 0.092 (−0.005, 0.19), P = 0.062]. Exploratory voxel-based analysis revealed an association between postural control and right hemispheric cholinergic network related to visual-vestibular integration and self-motion perception. High anti-cholinergic burden predicted postural control impairment in a manner dependent on right hemispheric cortical cholinergic integrity [β = 0.34 (0.065, 0.61), P < 0.01].
Our findings advance the perspective that cortical cholinergic system might play a role in supporting postural control after nigro-striatal dopaminergic losses in Parkinson’s disease. Failure of cortex-dependent visual-vestibular integration may impair detection of postural instability in absence of reliable proprioceptive cues. Better understanding of how the cholinergic system plays a role in this process may augur novel treatments and therapeutic interventions to ameliorate debilitating symptoms in patients with advanced Parkinson’s disease.
Introduction
Freezing of gait (FoG) is one of the most debilitating dopamine-refractory mobility disturbances in Parkinson’s disease (PD), leading to functional disability and lower quality of life in advanced stages of the disease. FoG is a major cause of falls which may result in increased morbidity and mortality.1 Despite its high clinical relevance, the pathophysiology of FoG largely remains an enigma.2 Postural instability is another dopamine-refractory symptom of PD associated with falls. Despite the common co-occurrence of these two symptoms, there is little agreement about how these are related.3 Theoretical discourse posits that either FoG is a consequence of postural instability due to behavioural interference (or vice versa), or they are not causally related to one another, instead sharing a common pathological underpinning (see Supplementary material, section 1, for illustration).4 Recent findings on postural stability in PD seem to lend support towards the shared pathology hypothesis. For example, increased postural sway has been associated with decreased thalamic cholinergic innervation in PD.5 Previous research has also found that PD fallers have reduced cortical and thalamic acetylcholinesterase hydrolysis rates as compared to non-fallers with PD.6 Furthermore, patients with PD who exhibit FoG (freezers) were more likely to fail condition 4 of the modified Romberg sensory conflict test (a test of sensory organization), which demands efficacious utilization of vestibular sensory information to maintain postural stability when proprioceptive information becomes unreliable.7 Accordingly, loss of cholinergic integrity within regions associated with performance on the Romberg 4 sensory conflict condition was observed among freezers as compared to non-freezers.8 Group differences in cholinergic innervation between freezers and non-freezers and between fallers and non-fallers have also been reported.9
Such evidence suggests that the shared cholinergic pathology underlying both FoG and postural instability might be mediated through a disruption in multisensory-motor and cognitive processing. Although the modified Romberg sensory conflict test is a reliable clinical tool for isolating the contributions of the vestibular system to postural stability, computerized posturography during the sensory organization test (SOT) offers greater flexibility, since it can better isolate the contribution of other sensory input streams (visual and proprioceptive) using centre of pressure (COP) measures. This will allow more sensitive detection of the role of multi-sensory integration during postural control than the dichotomized success/failure outcome measure given by the modified Romberg test. Prior research on computerized posturography among PD freezers successfully demonstrated a relative deficit in vestibular, proprioceptive, and visual processing associated with postural instability among freezers as compared to non-freezers.10 The primary dynamic posturography outcome measures in that study were based on the equilibrium score, which gives a general estimate of postural efficacy.10 Although this measure of balance is well-validated and accepted,10 there are recent advances in algorithms used to quantify features of postural control during quiet standing, some of which might illuminate how postural strategy differs in freezers.
The primary purpose of the study is to determine whether any of the recently reviewed features of postural control11 differ among freezers as compared to non-freezers, and to elucidate how these novel measures might relate to the established computerized posturography measures associated with FoG in PD. We also investigated whether measures of postural control that differentiate freezers from non-freezers may be associated with brain cholinergic system changes that can be reliably assessed in cortical, thalamic, and limbic structures by acetylcholinesterase PET.
Materials and methods
Study procedures, methods, and consent
The medical ethics committee at the University of Michigan (Institutional Review Board number HUM00022832) approved this study, and informed consent was obtained in all study participants. Subjects were recruited from a PET clinical correlation study in patients with PD (ClinicalTrials.gov Identifier: NCT01565473). The diagnosis of PD was confirmed by the UK Parkinson’s Disease Society Brain Bank clinical diagnostic criteria and consistent with a typical pattern of nigrostriatal dopaminergic denervation on 11C-dihydrotetrabenazine vesicular monoamine type 2 PET imaging in all participants. Eligibility for this study was also limited to participants who had no evidence of contra-indication for MRI, did not meet the Movement Disorder Society (MDS) criteria for PD dementia,12 were not taking anti-cholinergic or cholinesterase inhibitor drugs, did not have a history of Meniere’s disease, or recent history of acute vertigo, indicative of benign positional vertigo.
Large fibre lower extremity vibratory sensory information was based on the degree of vibratory sensation detection threshold at the lateral malleolus (right and left averaged) using the biothesiometer device (Bio-Medical Instrument Co.), an electric tuning fork providing superior accuracy compared to a manual tuning fork in determining vibratory thresholds for peripheral neuropathy assessment, with higher scores indicating more severe peripheral neuropathy [mean ± standard deviation (SD): 29.02 ± 10.94 Hz; range: 11.3–50 Hz). Participants completed the MDS-revised Unified Parkinson’s Disease Rating Scale (MDS-UPDRS) Part III (mean ± SD 29.8 ± 11.8; range: 8–66) and Hoehn and Yahr staging (2.3 ± 0.46; range: 1–4). Participants also completed the Montreal Cognitive Assessment (MoCA) (25.7 ± 2.5; range: 15–30). The majority of participants were on dopaminergic medications. Mean levodopa equivalent dose (LED) was 628.5 ± 493.1 (range: 0–3180) mg.1311C-methyl-4-piperidinyl propionate PET was used to assess regional cerebral acetylcholinesterase hydrolysis. A non-invasive analysis for PET imaging data was employed as previously reported.14 The non-invasive analysis allows for reliable assessment of hydrolysis rates in the cortex, limbic system, and thalamus but not in high intensity regions, such as the basal ganglia or the hypothalamus.
Computerized posturography
Study participants completed the Equitest posture test platform SOT (NeuroCom© International, Inc.) while off dopaminergic medications. NeuroCom force platform is a valid and reliable tool to measure postural impairment in PD.15 The dynamic posturography SOT consists of six sensory testing conditions to assess the integration of proprioceptive, visual, and vestibular functions during upright standing. The various conditions examine the individual’s ability to maintain standing using combinations of unaltered, altered (sway-referenced), or missing visual and proprioceptive cues during testing sessions lasting 20 s with short breaks in between. In SOT1 and 2, the person stands quietly with eyes open (SOT1) and closed (SOT2). This establishes whether sway increases when visual cues are removed and determines how effectively the participant makes use of somatosensory input. In SOT3, the person stands with their eyes open; the visual surround is sway-referenced and visual cues are no longer accurate. In SOT4, the support surface is sway-referenced; somatosensory cues are no longer accurate. SOT5 is performed with eyes closed and a sway-referenced support surface. SOT5 is the vestibularly challenging condition because it determines how the person uses vestibular cues when visual cues are removed, and somatosensory cues are no longer accurate. In SOT6, the support surface and visual surround are simultaneously sway-referenced, which indicates if the person relies on visual cues even when they are no longer accurate. A diagram summarizing the meaning of all six SOT conditions can be found in Fig. 1. Specific details on mechanics of the NeuroCom© Equitest platform can be found in the Supplementary material, section 2.
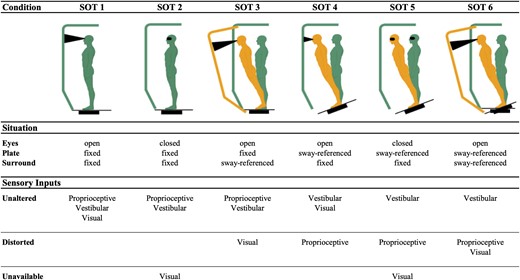
Summary of sensory organization test (SOT) conditions. Situation induced by each condition and the effect that it has on sources of sensory information for maintenance of balance are coupled with a visual representation to aid interpretation of our findings. Inspired by illustration in Huh et al.16 Created with BioRender.com
Equilibrium scores computed directly by the NeuroCom© Equitest platform were used as an established measure of overall postural efficacy. The equilibrium score is a unitless measure that roughly corresponds to the percentage of maximal angular bounds of stability (a range of 12.5 degrees) that is non-overlapping with the maximal sway angle (see Supplementary material, section 3, for details on equilibrium score calculation and interpretation). Openly available recently reviewed algorithms for reproducible calculation of COP features11 were applied to raw COP data from the NeuroCom© Equitest platform to generate a set of exploratory postural control features for a data-driven analysis.
Statistical analysis
Clinical and demographic group comparisons
Freezers and non-freezers were compared on a set of demographic, clinical, neuropsychological, and Parkinson’s specific features with membership in the freezer group defined as showing a score greater than 0 on the sum of MDS-UPDRS items 2.13 (freezing) and 3.11 (FoG). Demographic features included age, sex, years of education, weight, height, and handedness (one ambidextrous participant was recorded as left-handed). Clinical features included history of falls, balance impairment (Hoehn and Yahr score > 2.5), vibration sensitivity at the ankle as assessed by biothesiometer, and the presence of significant anti-cholinergic burden (ACB).17,18 ACB scores were calculated using the German ACB scale,18 as it is considered one of the highest quality scales for assessing ACB.17 Individuals were categorized as having either low ACB (score < 3) or high ACB (score ≥3), as it is recommended that patients switch to alternative drugs if they have an ACB score ≥3, and this is the suggested ceiling at which drug effects are considered to plateau.18 Neuropsychological features included overall cognition as assessed by MoCA scores, and executive function as assessed by seconds to complete the Delis–Kaplan Executive Function System Trail Making Test part IV (switching) minus part II (number tracing), an equivalent of Trail Making Test B − A. Parkinson’s-specific features included disease duration in years, levodopa equivalent dose calculation, total MDS-UPDRS III motor severity score (minus FoG related items), side of the body more affected by Parkinson’s symptoms, and integrity of nigro-striatal dopaminergic system as assessed by average striatal DTBZ-PET binding. The Mann–Whitney U-test was used to compare groups on numerical variables and chi-square test of independence was used for categorical variables. Group comparisons on covariates were two-tailed, and an α value of 0.2 was used as significance threshold to not miss any potentially important covariates for subsequent multivariate analyses. All group comparisons were performed with functions from SciPy, an open-source Python module.
Posturography statistics
Equilibrium scores across the six SOT conditions were visualized by group using notched box plots to identify the SOT condition that best differentiated freezers from non-freezers. Equilibrium score differences (contrasts) were calculated for a set of a priori SOT condition pairs to assess the effect of sensory manipulations on balance. SOT4 (eyes open, sway-reference platform) minus SOT1 (eyes open, fixed platform) was used to assess the effect of reliable proprioceptive cue loss on balance. SOT2 (eyes closed, fixed platform) minus SOT1 was used to assess the effect of visual cue loss on balance. SOT5 (eyes closed, sway-referenced platform) minus SOT1 was used to assess the effect of joint visual and proprioceptive cue loss on balance. Equilibrium score contrasts were visualized by group using notched box plot. Matplotlib and Seaborn open-source Python modules were used to create notched box plot visualizations.
A data-driven LASSO logistic regression was implemented to determine which of the 72 COP measures based on algorithms implemented by Quijoux et al.11 were predictive of freezing status under the SOT condition that best differentiated freezers from non-freezers on equilibrium score. The accuracy-maximizing L1-penalty hyperparameter (λ) was chosen based on leave-one-out cross-validation (LOO-CV). The accuracy-maximizing cutoff value was chosen based on maximal difference between true positive and false positive rate, and receiver-operating characteristics (ROC) of the resulting LASSO logistic regression model were computed. Standardized regression coefficients (β) of the fitted LASSO logistic regression model were examined, and the regressor with strongest absolute β coefficient was selected as the most robust novel COP predictor of freezing status for further analysis. COP paths for three participants most and least likely to exhibit freezing based on model predictions were visualized in 3D around the base of the support line to provide visual insight about multivariate differences in postural control between freezers and non-freezers. Cross-validated logistic LASSO regression implementation from open-source Python module Scikit-learn was used for the data-driven analysis of COP measures, and Matplotlib was used for COP path visualization.
The SOT condition pair found to best differentiate freezers from non-freezers on equilibrium score contrasts was used to calculate within-subject contrasts for the most robust novel COP predictor of freezing status and any other related COP measures on which the interpretation of the novel COP predictor was contingent. COP measures were z-transformed prior to difference score calculation and the difference scores were visualized using notched box plot. Most pronounced observed group differences on equilibrium score, equilibrium score contrast, and COP measure contrast box plots (least observed overlap in the notches) were tested with a two-tailed Mann–Whitney U-test, α = 0.05. To correct for family-wise error, the initial α was adjusted for the number of box plot-guided comparisons using conservative Bonferroni correction.
A hierarchical multiple logistic regression was carried out to assess relative contributions of equilibrium score contrast and novel COP measure contrast towards explaining the variance in FoG status. A likelihood ratio test was performed to compare goodness-of-fit between the equilibrium score contrast model and the null model, and between novel COP measure nested model and the equilibrium score contrast model. Standardized regression coefficients (β) and model coefficients of determination (R2) were used to interpret the relationship between established and novel posturography predictors of FoG. The Statsmodels open-source Python module was used for fitting all multivariate statistical models and functions from the SciPy module were used to perform likelihood ratio test model comparisons.
Neuroimaging statistics
An exploratory voxel-wise mass-univariate correlation analysis was conducted, predicting the novel COP measure contrast obtained in posturography analyses from acetylcholinesterase hydrolysis (PMP-k3) PET. Cluster-level correction for false discovery rate correction was applied to the resulting statistical parametric image19 and spatially contiguous clusters of significant association (minimal size in voxels = 50) were extracted and visualized in Montreal Neurological Institute space. Clusters localized to the cerebral cortex were projected and visualized on a high-resolution cortical surface mesh, to give better insight about their anatomical distribution. The Brodmann area cortical surface atlas was subsequently used to localize these clusters to specific cortical regions.20 Lastly, voxel counts and maximal t-values were tabulated by cluster and presented. Exploratory voxel-based findings were used to inform the region of interest definition for subsequent analyses. Prior to multivariate analyses, a univariate comparison of PMP-k3 in the identified region of interest between freezers and non-freezers, and fallers and non-fallers was conducted to assess for presence of group differences. The Nilearn open-source Python module was used to perform a mass-univariate voxel-wise analysis and cluster-level false discovery rate correction. For a detailed description of neuroimaging preprocessing see Supplementary material, section 4.
A more rigorous volume of interest (VOI) based multivariate analysis of postural control changes associated with FoG was carried out by fitting a hierarchical generalized linear model (GLM). Regressors were added to the model incrementally and retained if their presence significantly improved model fit to the distribution of the postural control regression and as assessed by likelihood ratio test for goodness of fit. The first regressor entering the model was averaged VOI PMP-k3 extracted from PET images in subject-native MRI space. The second regressor entering the model was FoG status, with model comparison effectively testing for independent contribution of FoG status to postural control. Third level regressor was an interaction between FoG status and VOI PMP-k3 which tested for potential dependence of cholinergic system regressor on FoG status. Subsequent regressors entered into the model included variables found to significantly differ between freezers and non-freezers on previously performed univariate group comparisons and lastly covariates not found to significantly differ between groups but contributions of which had to be accounted for on theoretical basis. Covariates that did not differ significantly between freezers and non-freezers but were included in the multivariate analysis to correct for any potential contribution that they might have on postural control were striatal dopaminergic integrity, overall cognition as assessed by MoCA score, executive function, age, and presence of high anti-cholinergic medication burden. Model fits were found to differ significantly if the P-value for the comparison fell below our significance threshold of α = 0.05.
Code and data availability
All the code used to perform group comparisons, posturography analyses, and neuroimaging statistics will be made publicly available on a GitHub repository upon publication or upon request during peer review process, for purposes of broader scientific scrutiny (URL: https://github.com/neuro-stivenr/neurocom-sda-fog-pmp). Data will not be made publicly available but will be provided upon reasonable request to the corresponding author.
Results
Clinical and demographic group differences
The current study presents data on 75 (freezers n = 16) out of 160 subjects originally enrolled, who met all eligibility criteria, were able to complete all six conditions of the NeuroCom© Equitest dynamic posturography SOT and also underwent 11C-methyl-4-piperidinyl propionate PET. Descriptive statistics and a complete set of group comparisons on covariates are presented in Table 1. Freezers were found to have fewer years of education (W = 647.5, P = 0.02), took higher doses of dopamine-replacement medications (W = 286.0, P = 0.02), experienced more severe motor symptoms (W = 260.5, P < 0.01), and tended to have greater frequency of clinical imbalance (χ2 = 3.85, P = 0.05).
Descriptive statistics and group comparisons between freezers and non-freezers
Variable . | Non-freezers (n = 59) . | Freezers (n = 16) . | Statistic . | P . |
---|---|---|---|---|
Age, years | 65.0 ± 10.0 | 67.5 ± 5.2 | W = 433.0 | 0.62 |
Education, years | 17.0 ± 4.0 | 15.5 ± 4.0 | W = 647.5 | 0.02* |
Weight, kg | 79.6 ± 22.0 | 79.9 ± 14.4 | W = 432.0 | 0.61 |
Height, cm | 174.0 ± 12.1 | 172.7 ± 10.5 | W = 534.0 | 0.43 |
Sex | F:14, M:45 | F:5, M:11 | χ2 = 0.08 | 0.77 |
Handedness | L:6, R:53 | L:4, R:12 | χ2 = 1.28 | 0.26 |
ACB cut-off | Low:43, High:16 | Low:9, High:7 | χ2 = 0.95 | 0.33 |
Clinical balance impairment | N:54, Y:5 | N:11, Y:5 | χ2 = 3.85 | 0.05* |
Clinical fall history | N:50, Y:9 | N:12, Y:4 | χ2 = 0.29 | 0.59 |
Vibration sensitivity, Hz | 27.3 ± 15.9 | 27.3 ± 15.4 | W = 486.5 | 0.86 |
MoCA score | 26.0 ± 3.0 | 27.0 ± 2.0 | W = 405.0 | 0.38 |
Trail Making Test (B − A) | 54.9 ± 39.1 | 48.4 ± 47.3 | W = 448.0 | 0.76 |
MDS-UPDRS III score | 25.5 ± 12.8 | 35.2 ± 15.2 | W = 260.5 | <0.01* |
PD duration, years | 4.0 ± 5.5 | 5.2 ± 5.0 | W = 417.5 | 0.48 |
More affected body side | Eq:2, L:21, R:36 | Eq:2, L:4, R:10 | χ2 = 2.39 | 0.30 |
LED, mg | 450.0 ± 417.0 | 685.0 ± 381.6 | W = 286.0 | 0.02* |
Striatal dopamine PET (DTBZ) | 1.9 ± 0.3 | 1.9 ± 0.4 | W = 442.0 | 0.70 |
Variable . | Non-freezers (n = 59) . | Freezers (n = 16) . | Statistic . | P . |
---|---|---|---|---|
Age, years | 65.0 ± 10.0 | 67.5 ± 5.2 | W = 433.0 | 0.62 |
Education, years | 17.0 ± 4.0 | 15.5 ± 4.0 | W = 647.5 | 0.02* |
Weight, kg | 79.6 ± 22.0 | 79.9 ± 14.4 | W = 432.0 | 0.61 |
Height, cm | 174.0 ± 12.1 | 172.7 ± 10.5 | W = 534.0 | 0.43 |
Sex | F:14, M:45 | F:5, M:11 | χ2 = 0.08 | 0.77 |
Handedness | L:6, R:53 | L:4, R:12 | χ2 = 1.28 | 0.26 |
ACB cut-off | Low:43, High:16 | Low:9, High:7 | χ2 = 0.95 | 0.33 |
Clinical balance impairment | N:54, Y:5 | N:11, Y:5 | χ2 = 3.85 | 0.05* |
Clinical fall history | N:50, Y:9 | N:12, Y:4 | χ2 = 0.29 | 0.59 |
Vibration sensitivity, Hz | 27.3 ± 15.9 | 27.3 ± 15.4 | W = 486.5 | 0.86 |
MoCA score | 26.0 ± 3.0 | 27.0 ± 2.0 | W = 405.0 | 0.38 |
Trail Making Test (B − A) | 54.9 ± 39.1 | 48.4 ± 47.3 | W = 448.0 | 0.76 |
MDS-UPDRS III score | 25.5 ± 12.8 | 35.2 ± 15.2 | W = 260.5 | <0.01* |
PD duration, years | 4.0 ± 5.5 | 5.2 ± 5.0 | W = 417.5 | 0.48 |
More affected body side | Eq:2, L:21, R:36 | Eq:2, L:4, R:10 | χ2 = 2.39 | 0.30 |
LED, mg | 450.0 ± 417.0 | 685.0 ± 381.6 | W = 286.0 | 0.02* |
Striatal dopamine PET (DTBZ) | 1.9 ± 0.3 | 1.9 ± 0.4 | W = 442.0 | 0.70 |
Descriptive statistics by group are presented as median ± interquartile range for numerical variables and as counts for categorical variables. Chi-square (χ2) statistic is given for categorical variables corresponding to χ2 test of independence and W statistic is given for numerical variables corresponding to Mann–Whitney U-test. Uncorrected two-tailed significant P-values are presented with an asterisk. ACB = anti-cholinergic burden; LED = levodopa equivalent dose; MDS-UPDRS = Movement Disorder Society-Revised Unified Parkinson’s Disease Rating Scale; MoCA = Montreal Cognitive Assessment.
Descriptive statistics and group comparisons between freezers and non-freezers
Variable . | Non-freezers (n = 59) . | Freezers (n = 16) . | Statistic . | P . |
---|---|---|---|---|
Age, years | 65.0 ± 10.0 | 67.5 ± 5.2 | W = 433.0 | 0.62 |
Education, years | 17.0 ± 4.0 | 15.5 ± 4.0 | W = 647.5 | 0.02* |
Weight, kg | 79.6 ± 22.0 | 79.9 ± 14.4 | W = 432.0 | 0.61 |
Height, cm | 174.0 ± 12.1 | 172.7 ± 10.5 | W = 534.0 | 0.43 |
Sex | F:14, M:45 | F:5, M:11 | χ2 = 0.08 | 0.77 |
Handedness | L:6, R:53 | L:4, R:12 | χ2 = 1.28 | 0.26 |
ACB cut-off | Low:43, High:16 | Low:9, High:7 | χ2 = 0.95 | 0.33 |
Clinical balance impairment | N:54, Y:5 | N:11, Y:5 | χ2 = 3.85 | 0.05* |
Clinical fall history | N:50, Y:9 | N:12, Y:4 | χ2 = 0.29 | 0.59 |
Vibration sensitivity, Hz | 27.3 ± 15.9 | 27.3 ± 15.4 | W = 486.5 | 0.86 |
MoCA score | 26.0 ± 3.0 | 27.0 ± 2.0 | W = 405.0 | 0.38 |
Trail Making Test (B − A) | 54.9 ± 39.1 | 48.4 ± 47.3 | W = 448.0 | 0.76 |
MDS-UPDRS III score | 25.5 ± 12.8 | 35.2 ± 15.2 | W = 260.5 | <0.01* |
PD duration, years | 4.0 ± 5.5 | 5.2 ± 5.0 | W = 417.5 | 0.48 |
More affected body side | Eq:2, L:21, R:36 | Eq:2, L:4, R:10 | χ2 = 2.39 | 0.30 |
LED, mg | 450.0 ± 417.0 | 685.0 ± 381.6 | W = 286.0 | 0.02* |
Striatal dopamine PET (DTBZ) | 1.9 ± 0.3 | 1.9 ± 0.4 | W = 442.0 | 0.70 |
Variable . | Non-freezers (n = 59) . | Freezers (n = 16) . | Statistic . | P . |
---|---|---|---|---|
Age, years | 65.0 ± 10.0 | 67.5 ± 5.2 | W = 433.0 | 0.62 |
Education, years | 17.0 ± 4.0 | 15.5 ± 4.0 | W = 647.5 | 0.02* |
Weight, kg | 79.6 ± 22.0 | 79.9 ± 14.4 | W = 432.0 | 0.61 |
Height, cm | 174.0 ± 12.1 | 172.7 ± 10.5 | W = 534.0 | 0.43 |
Sex | F:14, M:45 | F:5, M:11 | χ2 = 0.08 | 0.77 |
Handedness | L:6, R:53 | L:4, R:12 | χ2 = 1.28 | 0.26 |
ACB cut-off | Low:43, High:16 | Low:9, High:7 | χ2 = 0.95 | 0.33 |
Clinical balance impairment | N:54, Y:5 | N:11, Y:5 | χ2 = 3.85 | 0.05* |
Clinical fall history | N:50, Y:9 | N:12, Y:4 | χ2 = 0.29 | 0.59 |
Vibration sensitivity, Hz | 27.3 ± 15.9 | 27.3 ± 15.4 | W = 486.5 | 0.86 |
MoCA score | 26.0 ± 3.0 | 27.0 ± 2.0 | W = 405.0 | 0.38 |
Trail Making Test (B − A) | 54.9 ± 39.1 | 48.4 ± 47.3 | W = 448.0 | 0.76 |
MDS-UPDRS III score | 25.5 ± 12.8 | 35.2 ± 15.2 | W = 260.5 | <0.01* |
PD duration, years | 4.0 ± 5.5 | 5.2 ± 5.0 | W = 417.5 | 0.48 |
More affected body side | Eq:2, L:21, R:36 | Eq:2, L:4, R:10 | χ2 = 2.39 | 0.30 |
LED, mg | 450.0 ± 417.0 | 685.0 ± 381.6 | W = 286.0 | 0.02* |
Striatal dopamine PET (DTBZ) | 1.9 ± 0.3 | 1.9 ± 0.4 | W = 442.0 | 0.70 |
Descriptive statistics by group are presented as median ± interquartile range for numerical variables and as counts for categorical variables. Chi-square (χ2) statistic is given for categorical variables corresponding to χ2 test of independence and W statistic is given for numerical variables corresponding to Mann–Whitney U-test. Uncorrected two-tailed significant P-values are presented with an asterisk. ACB = anti-cholinergic burden; LED = levodopa equivalent dose; MDS-UPDRS = Movement Disorder Society-Revised Unified Parkinson’s Disease Rating Scale; MoCA = Montreal Cognitive Assessment.
Freezers exhibit less effective postural control in absence of reliable proprioceptive cues
A total of four group comparisons were performed for posturography-related variables, setting Bonferroni-adjusted α to 0.0125. Equilibrium scores by group across SOT conditions are presented in Fig. 2A. Freezers showed the greatest difference in performance relative to non-freezers on SOT4: 77.0 ± 11.5 (median ± interquartile range) and 82.0 ± 7.0, respectively. Mann–Whitney U-test confirmed that the distribution of equilibrium scores on SOT4 was significantly different between the two groups (W = 696.5, P < 0.01). Within-subject SOT condition contrasts are presented for loss of visual and proprioceptive cues either in isolation or jointly in Fig. 2B. The most pronounced group difference appears to be on the SOT4–SOT1 contrast (non-freezers: −9.0 ± 8.5, freezers: −18.0 ± 10.5). Mann–Whitney U-test confirmed that distribution of within-subject SOT4–SOT1 contrast was different between freezers and non-freezers (W = 723.5, P < 0.01).
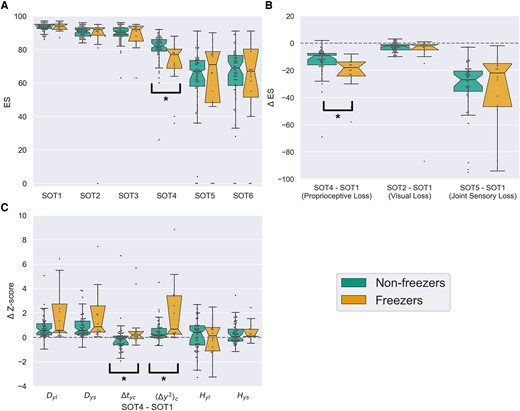
Box plot visualizations of posturography variables and sensory organization test (SOT) contrasts by group. (A) Notched box plot of equilibrium scores across SOT conditions by group. Notches represent 95% confidence intervals for the median and were computed with 10 000 bootstrapped samples. Maximal whisker size is 1.5 times the interquartile range. Outliers are represented as points beyond the whiskers. Significance of performed comparisons is indicated with a square bracket and asterisk. (B) Within-subject equilibrium score difference as a function of sensory cue loss from SOT manipulations. SOT4–SOT1 contrast assesses the effect of proprioceptive cue loss. SOT2–SOT1 contrast assesses the effect of visual cue loss. SOT5–SOT1 contrast assesses the effect of joint visual and proprioceptive cue loss. (C) Within-subject difference scores for z-transformed stabilogram diffusion analysis measures corresponding to SOT contrast related to loss of reliable proprioceptive cues. Only measures specific to anterior-posterior axis are presented. ⟨Δy2⟩c = critical mean square displacement in anterior-posterior axis; Δtyc = critical time interval in anterior-posterior axis; Dys = short term diffusion coefficient in y (AP) axis; Dyl = long term diffusion coefficient in y (AP) axis; Hys = short term scaling coefficient in y (AP) axis; Hyl = long term scaling coefficient in y (AP) axis.
Optimal λ value was determined by LOO-CV to be 0.23. Accuracy-maximizing cutoff for the resulting regularized logistic regression model was set to 0.35, with classification accuracy of 84%, sensitivity of 50%, and specificity of 93.2%. The resulting model had 6 retained regressors, with the strongest ones being a decrease in fractal dimension in anterior-posterior and medio-lateral axis (β = −0.145), an increase in total power spectrum density in the medio-lateral axis (β = 0.191), and an increase in critical mean square displacement in anterior-posterior axis (⟨Δy2⟩c) (β = 0.419). Detailed descriptions of all the retained regressors can be found in Quijoux et al.,11 but for the sake of parsimony we chose to focus on ⟨Δy2⟩c as the exploratory COP measure for further examination because its absolute β coefficient was higher than other regressors. 3D plots of COP paths for participants most and least likely to exhibit FoG based on whole model predictions are presented in Fig. 3, and the list of all retained regressors can be found in Supplementary material, section 5.
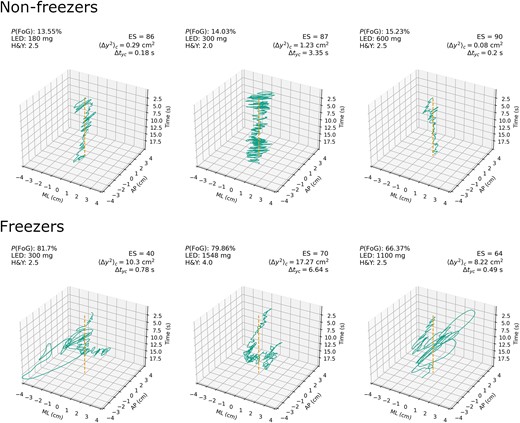
Raw stabilogram plots for sensory organization test 4 (SOT4) by group. Group membership, logistic LASSO model probability of FoG, LED, equilibrium score, critical mean square displacement in anterior-posterior axis on SOT4, and critical time interval in anterior-posterior axis on SOT4. The top row represents three participants least likely to have FoG based on logistic LASSO regression model predictions (true negatives). The bottom row represents three participants most likely to have FoG based on model predictions (true positives). Dashed line in the centre represents mean of COP point distribution, which gives a reference frame for examining sway around the base of support. ⟨Δy2⟩c = critical mean square displacement in y anterior-posterior axis; Δtyc = critical time interval in anterior-posterior axis; P(FoG) = model-predicted probability of FoG status. AP = anterior-posterior; ES = NeuroCom© Equitest equilibrium score; H&Y = Hoehn and Yahr (clinical postural stability score); LED = levodopa-equivalent dose; ML = medio-lateral .
Distributions of anterior-posterior axis stabilogram diffusion analysis measure contrasts from SOT4 to SOT1 are presented by group in Fig. 2C. Most pronounced shifts in distribution between groups appear on critical time interval (Δtyc) (non-freezers: −0.16 ± 0.73, freezers: 0.34 ± 0.7) and ⟨Δy2⟩c; non-freezers: 0.19 ± 0.58, freezers: 0.7 ± 3.05). Mann–Whitney U-test demonstrated a significant difference in distribution of both ⟨Δy2⟩c (W = 358.0, P < 0.01) and Δtyc (W = 329.0, P < 0.01) SOT4–SOT1 contrasts between freezers and non-freezers. Given the central importance of changes in Δtyc and ⟨Δy2⟩c that we observed as a function of sensory adaptation in postural control of freezers, idealized stabilogram diffusion plots across all six SOT conditions were generated by group to give insight into global patterns of changes in postural control between freezers and non-freezers that might have been missed by our primary posturography analyses focusing specifically on the loss of reliable proprioceptive cues (Fig. 4).
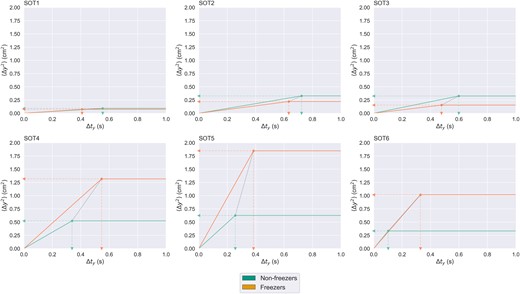
Idealized (based on critical point coordinates only) stabilogram diffusion plots by group across every SOT condition. The horizontal axis represents time intervals of varying length. The vertical axis represents average mean square displacement in anterior-posterior axis over all the time intervals of a given length. Critical point coordinates for each group are calculated by taking the median of critical time interval, Δtyc, and critical mean square displacement, ⟨Δy2⟩c, for a given SOT condition. Dashed arrows pointing to horizontal and vertical axes represent group medians of Δtyc and ⟨Δy2⟩c. Dotted line drawn between critical points represents separation of critical point between the groups. Δt = time interval in seconds; ⟨Δy2⟩ = mean square displacement in anterior-posterior axis; SOT(1–6) = various conditions of the sensory organization test.
First level hierarchical logistic regression model accounted for significantly more variance in freezing status than the null intercept model (χ2 = 6.56, P = 0.01, = 0.084), with decreases in equilibrium score as a function of proprioceptive cue loss associated with greater probability of freezing status [β = −0.68 (−1.25, −0.11), P = 0.019]. Second level hierarchical logistic regression model outperformed the first level model at accounting for variance in freezing status (χ2 = 7.75, P < 0.01, = 0.18), with increases in ⟨Δy2⟩c as a function of proprioceptive cue loss associated with greater probability of freezing [β = 0.89 (0.14, 1.63), P = 0.019]. Furthermore, the presence of the ⟨Δy2⟩c contrast regressor in the model tended to weaken the regression coefficient magnitude of the equilibrium score contrast regressor [β = −0.41 (−0.99, 0.18), P = 0.17], which suggests that change in ⟨Δy2⟩c shares a lot of captured variance in freezing status with change in equilibrium score, but goes beyond the shared portion of variance as supported by a greater coefficient of determination in the second level model.
Right-Hemisphere cortical PMP-k3 associates with postural control characteristics of freezers
Voxel-wise correlation between changes in ⟨Δy2⟩c as a function of reliable proprioceptive cue loss and brain acetylcholinesterase hydrolysis rates (PMP-k3) yielded 10 clusters of significant association after false discovery rate correction, with most significant clusters localized in the right hemisphere (Fig. 5). These clusters can be grouped based on their functional and anatomical significance (see Table 2 for cluster definitions and statistics). Clusters 1 and 4 represent multi-modal sensory regions including supramarginal gyrus, angular gyrus, and posterior superior temporal gyrus. Clusters 3 and 8 represent visual associative cortices along the ventral and dorsal visual pathway respectively. Clusters 2, 6, and 7 represent secondary motor cortices (premotor and supplementary motor cortex) and a prefrontal cortical region. Clusters 5 and 9 form the only bilateral grouping and represent primarily inferior frontal gyrus. Cluster 10 is the only one localized to the left hemispheres and includes ventral temporal cortices. Summary statistics on clusters of significant association are presented in Table 2. Cortical surface projection of retained clusters (Fig. 6) reveals a lateralized pattern of association between PMP-k3 and ⟨Δy2⟩c SOT4–SOT1 contrast in the right hemisphere, concentrated around the Sylvan fissure, parietal operculum, and temporo-parietal junction. The composite VOI for subsequent analyses was identified as right hemisphere cerebral cortex. Group comparison of right hemisphere cerebral cortex PMP-k3 distributions yielded no significant group differences on FoG status (W = 345.0, P = 0.10), with a trend towards relative preservation of cholinergic integrity in right cerebral hemisphere of freezers. Comparison between fallers and non-fallers was also non-significant (W = 499.0, P = 0.18), with a trend towards lower cholinergic integrity in the right cerebral hemisphere of fallers (see Supplementary material, section 6 for box plots).
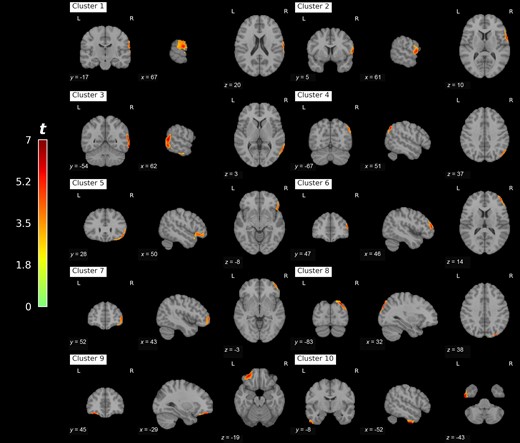
Clusters of false discovery rate corrected correlation between PET acetylcholinesterase hydrolysis rates and change in anterior-posterior critical mean square displacement as a function of reliable proprioceptive cue loss during postural control. MNI space coordinates are provided for cluster centroids. L = left; R = right.
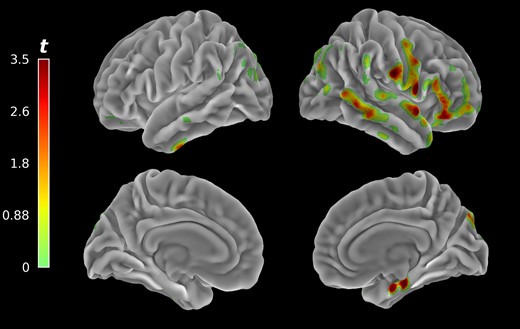
Voxel-wise clusters of significant associations between 11C-methyl-4-piperidinyl propionate PET acetylcholinesterase hydrolysis rates projected unto cortical surface mesh. Associated cholinergic system changes were predominantly present in right hemispheric cortical structures related to visual-vestibular integration and self-motion perception.
Descriptions of retained clusters from 11C-methyl-4-piperidinyl propionate PET correlational analysis
Cluster . | Brodmann areas . | Number of voxels . | Maximal t-value . |
---|---|---|---|
1 | Right BA40/22/2 | 2081 | 5.66 |
2 | Right BA44/6/3/1 | 2043 | 5.61 |
3 | Right BA21/37 | 7018 | 6.44 |
4 | Right BA39 | 1567 | 6.05 |
5 | Right BA47/45 | 3209 | 5.66 |
6 | Right BA46 | 1592 | 5.40 |
7 | Right BA10 | 1740 | 4.85 |
8 | Right BA19/7 | 2313 | 6.51 |
9 | Left BA47 | 1406 | 6.26 |
10 | Left BA20 | 1376 | 6.85 |
Cluster . | Brodmann areas . | Number of voxels . | Maximal t-value . |
---|---|---|---|
1 | Right BA40/22/2 | 2081 | 5.66 |
2 | Right BA44/6/3/1 | 2043 | 5.61 |
3 | Right BA21/37 | 7018 | 6.44 |
4 | Right BA39 | 1567 | 6.05 |
5 | Right BA47/45 | 3209 | 5.66 |
6 | Right BA46 | 1592 | 5.40 |
7 | Right BA10 | 1740 | 4.85 |
8 | Right BA19/7 | 2313 | 6.51 |
9 | Left BA47 | 1406 | 6.26 |
10 | Left BA20 | 1376 | 6.85 |
BA = Brodmann area.
Descriptions of retained clusters from 11C-methyl-4-piperidinyl propionate PET correlational analysis
Cluster . | Brodmann areas . | Number of voxels . | Maximal t-value . |
---|---|---|---|
1 | Right BA40/22/2 | 2081 | 5.66 |
2 | Right BA44/6/3/1 | 2043 | 5.61 |
3 | Right BA21/37 | 7018 | 6.44 |
4 | Right BA39 | 1567 | 6.05 |
5 | Right BA47/45 | 3209 | 5.66 |
6 | Right BA46 | 1592 | 5.40 |
7 | Right BA10 | 1740 | 4.85 |
8 | Right BA19/7 | 2313 | 6.51 |
9 | Left BA47 | 1406 | 6.26 |
10 | Left BA20 | 1376 | 6.85 |
Cluster . | Brodmann areas . | Number of voxels . | Maximal t-value . |
---|---|---|---|
1 | Right BA40/22/2 | 2081 | 5.66 |
2 | Right BA44/6/3/1 | 2043 | 5.61 |
3 | Right BA21/37 | 7018 | 6.44 |
4 | Right BA39 | 1567 | 6.05 |
5 | Right BA47/45 | 3209 | 5.66 |
6 | Right BA46 | 1592 | 5.40 |
7 | Right BA10 | 1740 | 4.85 |
8 | Right BA19/7 | 2313 | 6.51 |
9 | Left BA47 | 1406 | 6.26 |
10 | Left BA20 | 1376 | 6.85 |
BA = Brodmann area.
Right cortical PMP-k3, freezing of gait, and motor severity independently predict postural efficacy
Distribution of ⟨Δy2⟩c SOT4–SOT1 contrast was plotted as a histogram (Supplementary material, section 7), and determined to be approximately Gamma-distributed, so Gamma family GLM with log link function was chosen. Right cortical PMP-k3 accounted for more variance in response distribution than the null intercept model (χ2 = 20.214, P < 0.001, = 0.114). Independent effect of FoG status model had significantly better fit than the right cortical PMP-k3 model (χ2 = 19.769, P < 0.001, = 0.310). The right cortical PMP-k3 by FoG status model was a significantly better fit than the FoG status independent model (χ2 = 4.426, P = 0.035, = 0.355). Motor severity of disease was added as the next regressor and significantly improved model fit (χ2 = 7.285, P = 0.007, = 0.426), furthermore driving the right cortical PMP-k3 by FoG status interaction term non-significant [β = 0.17 (−0.10, 0.45), P = 0.22]. An additional model comparison was performed between the original motor severity model and the reduced model without an interaction term, which revealed that the right cortical PMP-k3 by FoG status interaction term did not contribute to model prediction of response distribution (χ2 = 1.454, P = 0.23), so it was subsequently dropped to maintain model parsimony. Addition of subsequent covariates found to differ significantly between groups, including LED (χ2 = 0.153, P = 0.7), clinical imbalance (χ2 = −0.235, P = 1.0), and education years (χ2 = −0.189, P = 1.0) did not improve prediction so none of them were retained in the model. Addition of striatal dopaminergic integrity PET (χ2 = −0.24, P = 1.0), MoCA score (χ2 = −0.307, P = 1.0), and executive function (χ2 = 0.412, P = 0.52) did not improve prediction so none of them were retained in the model. Addition of age significantly improved prediction (χ2 = 4.315, P = 0.038, = 0.466), so it was retained. Resulting model included independent contributions of FoG status [β = 0.37 (0.088, 0.65), P < 0.01], right cortical PMP-k3 [β = 0.13 (0.021, 0.24), P = 0.019], motor severity of disease [β = 0.17 (0.057, 0.28), P < 0.01], and age [β = 0.097 (−0.01, 0.20), P = 0.075].
Effect of anti-cholinergic burden on balance depends on cholinergic integrity of the right cortex
Presence of high ACB contributed to prediction accuracy (χ2 = 4.390, P = 0.036, = 0.521), though the model coefficient for the high ACB term was only marginally significant [β = 0.20 (−0.015, 0.43), P = 0.068]. We suspected that an interaction might be present between right cortical PMP-k3 and high ACB, so an additional model comparison was performed between the independent effect of high ACB and the interaction model. Model comparison revealed that right cortical PMP-k3 and high ACB interaction model accounted for variance in response distribution significantly better than the independent effect of ACB model (χ2 = 6.988, P = 0.008, = 0.594). In the resulting model, coefficients for independent effect of FoG status [β = 0.28 (0.021, 0.54), P = 0.034] and motor severity [β = 0.16 (0.062, 0.26), P < 0.01] retained their significance, while age remained just above significance threshold [β = 0.092 (−0.005, 0.19), P = 0.062]. Marginal effects of right cortical PMP-k3 [β = 0.072 (−0.033, 0.18), P = 0.18] and high ACB [β = 0.17 (−0.036, 0.38), P = 0.10] were not statistically significant, but their interaction term was [β = 0.34 (0.065, 0.61), P = 0.015]. Detailed visualization of model fit, coefficients at every step of the hierarchical GLM fitting process, and final model normality of deviance residuals and multicollinearity diagnostics can be found in Supplementary material, section 7. Deviance residuals were approximately normally distributed, no excessive correlation was detected between the regressors on a Spearman correlation matrix, and variance inflation factor did not exceed 1.5 for any of the regressors suggesting minimal presence of multicollinearity.
Discussion
Proprioceptive dependence of postural control among freezers
Our analysis of balance performance under sensory perturbation revealed that differences between freezers and non-freezers were most pronounced when proprioceptive cues are rendered unreliable (SOT4), and participants must rely on some combination of visual and vestibular cues to maintain balance. Our set of within-subject contrasts between SOT conditions revealed loss of proprioceptive cues, SOT4–SOT1, to be the contrast that maximizes the distinction between freezers and non-freezers, with freezers showing greatest within-subject decrease in balance performance in the absence of reliable proprioceptive cues. These findings agree with prior posturography results showing poorer equilibrium scores for freezers under SOT416 and greater magnitude of sway during balance on a foam surface with eyes open, a condition analogous to SOT4.21
According to foundational studies in sensory reweighting during postural control, the proprioceptive system produces the greatest contribution to balance during quiet standing under optimal conditions relative to visual and vestibular systems.22 However, when proprioceptive cues are rendered less reliable, as might be the case under inclined or uneven terrain, greater relative contributions of visual and vestibular systems are needed to offset this loss of sensory input for postural control. Other studies point towards slower reweighing of proprioceptive cues with gradual decreases in their reliability due to external perturbation in patients with PD as compared to control participants.23 Our findings taken together with prior work on sensory reweighing during postural control in PD point towards critical dependence on reliable proprioceptive cues, which might be present before onset of advanced PIGD symptoms such as FoG, but that is more exacerbated in freezers. Furthermore, differences between freezers and non-freezers appeared more pronounced when visual cues were unaltered as opposed to conditions where visual cues were rendered unreliable (i.e. sway-referencing visual surround) or absent (i.e. eyes closed). In the latter two cases, balance was roughly equivalent between the two groups, suggesting that poorer balance in freezers might be rooted in faulty visual-vestibular interaction rather than failure of any single sensory system.
Postural control changes under sensory manipulation in freezers and non-freezers
After identifying SOT4 as the condition where postural stability is most affected among freezers, we set out to characterize precise features of postural control that differentiate freezers from non-freezers in the absence of reliable proprioceptive cues. Our data-driven approach yielded critical mean square displacement in anterior-posterior axis, ⟨Δy2⟩c, as the most robust predictor of freezing status in the absence of reliable proprioceptive cues. Previous findings pertaining to ⟨Δy2⟩c during quiet standing include greater critical displacement in young adults in the absence of visual cues,24 in the older adults as compared to young adults,25 in older adults who are more susceptible to injurious falls as compared to those who are not,26 and in patients with PD compared to NC.27 The within-subject contrast between conditions 4 and 1 revealed freezer-specific increases in ⟨Δy2⟩c and critical time interval, Δtyc. Our hierarchical regression model predicting freezing status from equilibrium score and ⟨Δy2⟩c contrast revealed that the variance in freezing status accounted for by equilibrium score contrast is a subset of variance accounted for by the ⟨Δy2⟩c contrast, which suggests that stabilogram diffusion analysis measures of postural control likely capture the same underlying construct of postural instability as equilibrium score and furthermore might be more sensitive at detecting features of postural instability which are more pronounced among freezers.
Stabilogram plots for three non-freezers and three freezers appear to show that differences in Δtyc and ⟨Δy2⟩c between the two groups have an observable impact on pattern of COP sway under SOT4 (Fig. 3). Freezers appear to exhibit more exaggerated sway trajectories and a more pronounced low-frequency oscillation of the COP around the base of support in anterior-posterior axis. Non-freezers appear to exhibit a more pronounced high-frequency oscillation in anterior-posterior axis and maintain their COP within a relatively narrow area around the base of support. While column one and three fit the pattern described above well, column two shows evidence of qualitative and quantitative deviation from that pattern. Freezer stabilogram in column two does not appear to exhibit ballistic trajectories and has a more pronounced high-frequency component of sway, but still exhibits a characteristic pattern of low-frequency oscillation of COP around the base of support. Non-freezer stabilogram in column two appear to have a higher amplitude of high-frequency oscillation as compared to other non-freezers. Both of participants in column two appear to demonstrate different clinical balance impairment score and distinct differences in Δtyc and ⟨Δy2⟩c, which might be contributing factors to these observed differences. Future research on postural control of Parkinson’s freezers might benefit from the use of clustering algorithms to more effectively detect the multivariate patterns of postural control that we observed in our stabilogram plots, since differences on individual measures of COP sway may not fully capture the motifs that can be observed in raw stabilogram visualizations.
Idealized stabilogram diffusion plots across all six SOT conditions (Fig. 4) reinforce many of the conclusions that we came to in our primary analyses of postural control among freezers and non-freezers. Critical point coordinates among freezers and non-freezers appear to follow largely the same trend as a function of visual system manipulations under reliable proprioceptive cues and remained largely in vicinity of each other, suggesting that capacity for reconciling conflict between proprioception and other sensory systems is not more impaired among freezers as compared to non-freezers. In absence of reliable proprioceptive cues, freezers appear to consistently demonstrate greater Δtyc than non-freezers, with greater difference as a function of sensory conflict (SOT6 > SOT4 > SOT5). Difference in ⟨Δy2⟩c between freezers and non-freezers also appear more pronounced in absence of proprioceptive cues, with greatest difference observed under SOT5, which requires efficacious use of vestibular information for balance. Among freezers, difference in ⟨Δy2⟩c between SOT4 (reliable visual cues) and SOT6 (sway-referenced visual cues) appears more modest, suggesting that presence rather than informativeness of visual cues might play a stronger role for effective postural adjustments in freezers. No firm conclusions can be drawn from our idealized stabilogram diffusion plots due to their exploratory nature, so the trends we observed remain to be falsified or confirmed by future research.
Postural control changes under visual-vestibular conflict
Critical time interval, Δtyc, is the length of time over which a single epoch of postural control transpires, in other words, the time it takes for sensory information to be received, integrated with other modalities, and acted upon via top-down postural control mechanisms. ⟨Δy2⟩, is the amount of sway that occurs on average over each epoch of postural control. This account is based on Collins and Luca’s original interpretation of stabilogram diffusion characteristics (detailed explanation provided in Supplementary material, section 8), wherein displacements of the COP are largely driven by destabilizing fluctuation over shorter intervals of time and by stabilizing top-down postural adjustments over longer intervals of time.28 Increases in Δtyc can thus be conceived of as consequence of greater processing demand on the postural control system, which might be caused by ambiguous or incongruent sensory inputs. Increases in ⟨Δy2⟩c can be conceived of as net decrease in effectiveness of postural adjustments for maintenance of balance. Given that pronounced increases in both parameters occur among freezers in absence of reliable proprioceptive cues, we conclude that freezers experience greater difficulty with reconciling conflict between visual and vestibular sensory cues, possibly due to greater impairment in vestibular processing, which leads to reduced efficacy of postural adjustments.
Valid criticisms have been levied against Collins and Luca’s account that stabilogram diffusion characteristics are best explained by a superposition of open and closed-loop control dynamics as a function of time interval.28 Peterka posits that closed-loop control dynamics are sufficient to yield physiologically plausible stabilogram diffusion characteristics, and thus should be preferred due to greater parsimony.29 In their model, angular error signal is defined as deviation from upright stance, and a central controller (i.e. the brain) continuously generates postural adjustments via ankle torque in proportion to sway angle, sway angular velocity, and the integral of sway angle. Based on their simulation results,29 decreased response to the sway angle and velocity, along with increased response to the integral of sway angle, might account for the pattern of changes in stabilogram diffusion characteristics that we observed in our sample as a function of proprioceptive cue loss. Proprioceptive cues provide information about body position, including sway angle, while the visual and vestibular systems provide information about velocity. When on a sway-referenced surface, proprioceptive information about body tilt is distorted, leading to down-weighting of proprioceptive cues and a subsequent decrease in response to sway angle. Visual and vestibular systems both play a role in perception of bodily velocity, so failure to reconcile sensory conflict between them might lead to loss of precision in estimates of sway angular velocity and a subsequent attenuation of postural responses related to sway angular velocity. Thus, our findings can reasonably be interpreted as a failure to effectively reconcile conflict between visual and vestibular sensory streams in absence of reliable proprioceptive cues within both the original framework for interpreting stabilogram diffusion characteristics28 and one of the better-known alternative interpretations.29
Right cortical cholinergic network related to sensory integration and conflict monitoring
Our correlational analysis of acetylcholinesterase PET and postural control features most pronounced among freezers revealed that failure to efficaciously integrate visual and vestibular information for maintenance of balance correlates with a cholinergic network of right hemisphere predominant regions. The strongest cluster of association observed in our analysis based on the number of significant voxels (Cluster 3) contains Brodmann area 37, which is the human homologue of primate middle superior temporal (MST) area.30 It contains separate populations of neurons attuned selectively to either visual or vestibular motion stimuli, with dynamic changes in the weighing of each modality on the level of population dynamics as a function of stimulus intensity and reliability.31 It is thought to subserve a system for quick disentanglement of body motion and visual field motion through joint processing of velocity information contained in optic flow and velocity information integrated from vestibular acceleration inputs.30 Under reliable visual inputs, its population dynamics primarily reflect processing of visual signals.30
BA19 and BA7, which comprise the second strongest cluster of lateralized association in our analysis (Cluster 8), contain the lateral intraparietal area (LIP). Whereas area MST is the primary point of visual and vestibular convergence for processing of velocity, the LIP serves the same role for inertial processing.32 It receives inputs regarding acceleration from vestibular cortices and inputs regarding velocity from area MST.30 Integration of visual and vestibular information happens with a temporal offset of about 400 ms, with the visual signal lagging behind,33 which suggests a period of time when velocity-related evidence accumulates in the LIP prior to integration with vestibular afferents for multi-modal representation of bodily acceleration. This would be consistent with psychophysical evidence that visual acceleration perception likely does not occur directly, as with the vestibular otolith organ, but rather as a temporal differentiation of velocity derived from optic flow.34 Interestingly, informativeness of visual inputs during postural control modulate postural response to galvanic vestibular stimulation (GVS) only at around 400 ms,35 which is consistent with the window of integration between vestibular and visual cues in the LIP. Presence rather than absence of visual cues, however, had an immediate effect on postural response to GVS.35 Downstream regions in the parietal stream of self-motion perception processing observed in our correlational analysis include BA39 (supramarginal gyrus) and BA40 (angular gyrus), where information about velocity and acceleration is mapped unto a common bodily reference frame.30 Increased theta and delta band activity in angular gyrus has been observed during perception of self-acceleration, with weaker increases when the head or eyes were not aligned with direction of motion.36
The blood oxygen level-dependent response in inferior frontal gyri have been previously associated with joint visual and vestibular stimulation,37 and EEG activity during postural control under proprioceptive perturbation with open eyes is dominated by a network that involves inferior frontal gyri, sensory-motor frontal cortices, prefrontal cortices, and visual cortices, many of which heavily overlap with our acetylcholinesterase PET findings.38 Importantly, networked activity between inferior frontal gyri, sensory-motor cortices, and subthalamic nucleus have been demonstrated during post-error slowing during reaction time task in patients with PD.39 Greater engagement of these frontal structures during postural control might be related to reconciliation of conflicting sensory inputs and inhibition of postural control responses under unreliable sensory conditions. FoG, by extension of the same principle, might be an extreme case under which all top-down motor outputs are blocked due to extreme uncertainty under conditions of high sensory conflict.
Right hemispheric cholinergic system contributes to multi-sensory top-down control of balance
Previous studies from our group demonstrated that reduced cholinergic integrity in the thalamus, but not the cortex, associates with greater sway speed (poorer postural control) under SOT1, 2, 3 (platform fixed, eyes open, closed, or sway-referenced vision) and 5 (platform sway-referenced, eyes-closed).5 With conditions 1, 2, 3 the platform is fixed, meaning proprioceptive control of balance predominates22 and effective postural control might depend on effective reweighting of sensory afferents in the thalamus based on their correspondence with the proprioceptive bodily reference frame. Performance on SOT5 mostly reflects effective utilization of vestibular information for balance, shown to be impaired among freezers,7 and among PD patients with clinical postural instability as compared to controls.40 Strongest cholinergic correlate of impairment under vestibular challenging condition of the Romberg test was the medial geniculate nucleus of the thalamus.8 Thus, successful performance on Romberg 4 (closed eyes, foam surface) and SOT5 (closed eyes, sway-referenced surface), might be primarily a function of effective gain modulation of vestibular afferents via thalamic cholinergic circuitry.
In the present study, we chose to focus on SOT4 because our primary aim was to examine how postural control might differ among freezers, and SOT4 showed greatest contrast in postural performance between freezers and non-freezers. Compared to SOT1, 2, 3 and 5, condition 4 presents the greatest potential for sensory conflict, since generally predominating contributions of the proprioceptive system are rendered unreliable and can no longer be used as a stable reference frame. Unlike in SOT5, postural control of balance is not driven by a single modality, since visual and vestibular systems provide complementary information about velocity and inertia in a head-centred reference frame.30 Thus, failure to effectively balance under SOT4 likely reflects impaired capacity to appropriately weigh contributions from two parallel sensory input streams in a head-centred reference frame in absence of reliable sensory inputs from the body-centred reference frame—which might depend on the higher-order multimodal integration capacities of the cerebral cortex.
Lateralization of our cholinergic PET findings to the right cortical hemisphere contributes to a growing body of evidence for its preferential involvement in cortical control of posture. Studies of lateralized brain damage effects on balance show that right hemispheric lesions are more associated with anterior-posterior sway,41 and that patients with right hemispheric lesions are less able to adapt postural perturbation and are particularly vulnerable to loss of reliable proprioceptive inputs.42 Cholinergic system signaling in the right prefrontal cortex also has greater association with cue detection mechanisms,43 a function that might be relevant for cortical detection of postural instability prior to engagement of top-down compensatory responses. Cortico-cortical vestibular network is more strongly expressed in the right hemisphere,44 which makes it a more likely contender for cortical site of visual-vestibular integration. Lastly, recent review of neuroimaging studies of FoG expressed that accumulating evidence points towards right hemispheric lateralization of increased white matter pathology, structural, and functional connectivity changes among freezers.45
A trend for relative preservation of right cortical cholinergic integrity among freezers and a trend for relative loss of right cholinergic integrity among fallers was observed in our sample, which is in agreement with our previous findings. In one of our previously published studies of cholinergic system changes among Parkinson’s fallers and freezers no significant difference was shown on overall neocortical vesicular acetylcholine transporter binding between freezers and non-freezers, though more granular differences were observed on the voxel-based analysis specifically within limbic cortices.9 There was a stronger overlap between our present finding and the pattern of cholinergic losses observed in participants with a history of falls as opposed to those without, specifically the right lateralized losses in premotor, lateral temporal, temporopolar, lingual cortices, and lateral geniculate nucleus of the thalamus in conjunction with bilateral prefrontal cortices.9 Reason for lack of significant differences in our present sample might be lower disease severity and lower sensitivity of acetylcholinesterase PET radioligand as compared to vesicular acetylcholine transporter radioligand. Furthermore, the most pronounced differences in cholinergic innervation between freezers and non-freezers were observed in the striatum,9 a region we were not able to examine due to the limitations of acetylcholinesterase PET.
Despite no significant differences in right hemispheric cholinergic integrity among freezers and non-freezers within our sample, we were able to observe significant differences in effectiveness of their postural control under high visual-vestibular integration demand. Our generalized linear model demonstrated that deficits in postural control as a function of reliable proprioceptive cue loss is predicted by motor severity of disease and FoG status independently of one another, suggesting that impaired postural control arises from joint contribution of overall disease progression and freezer-specific pathology. Moreover, we were able to show that right cortical hemisphere cholinergic system indeed plays a role in postural control under high visual-vestibular integration demand, since the balance-impairing effect of ACB depended on integrity of right hemisphere cholinergic system. Thus, at least in earlier stages of PD progression, both freezers and non-freezers might rely on the relatively preserved right cerebral cholinergic system to maintain postural stability under challenging conditions. Loss of cholinergic integrity in those regions later in the disease as observed among fallers in our previous study9 might plausibly lead to greater postural instability among both freezers and non-freezers, with freezer-specific losses in striatal cholinergic signaling9 interacting with cortico-thalamic cholinergic system changes associated with falling to yield most severe manifestation of postural instability and the additional freezing symptoms.
Effect of anticholinergic and cholinergic medications on postural control
Our findings suggest that suppression of right cortical cholinergic system by ACB led to greater imbalance in PD patients, an effect that was independent of motor disease severity and FoG status. Clinical takeaway from this finding is that ACB should be closely monitored by primary care providers for individuals with PD, since their risk of imbalance and consequent falls is already amplified by their motor symptoms, and among freezers there is an additional freezer-specific postural control impairment. Our findings are consistent with the results of a large-scale study of ACB and negative health outcomes in the PD population, which showed that greater ACB associated with greater incidence of hospitalization due to fractures.46 Thus, high ACB poses an additional, potent risk factor for imbalance and should be minimized when possible.
Conversely, pro-cholinergic interventions have shown some promise, albeit with heterogenous consistency, at improving gait and balance symptoms of PD. One study showed specific improvement in balance performance on NeuroCom SOT4 after administration of donepezil, an acetylcholinesterase inhibitor.47 Another acetylcholinesterase inhibitor, rivastigmine, was shown to improve postural control under SOT5, during which vestibular inputs primarily drive postural control.48 Relatively recent meta-analysis of findings from studies not mentioned here, however, found mild to no improvement in balance as a function of acetylcholinesterase inhibitor treatment.49 However, a recent study examining nicotinic receptor agonist treatment showed significant improvement in frequency of falls and severity of FoG with mild improvement in levodopa-induced dyskinesia.50 Greater effectiveness of nicotinic acetylcholine agonist treatment might in part be due to its selective action, since short-duration optogenetic activation of striatal cholinergic interneurons enhanced expression of levodopa-induced dyskinesia, an effect dependent on mAChR, whereas their long-term stimulation decreased expression of levodopa-induced dyskinesia, an effect that was dependent on nAChR and presynaptic neuroplasticity.51 Nicotine treatment exhibited a similar efficacy to long-term stimulation.51 Conversely, acetylcholinesterase inhibitors would lead to a non-specific increase in cholinergic signaling, both nicotinic and muscarinic, which may account for heterogeneity in their effectiveness. Whether pro-cholinergic intervention depends on specific forms of cholinergic signaling in the cortex to improve symptoms remains to be elucidated by future research.
Dopaminergic therapy and postural control
For present work, we chose to perform all testing in the dopaminergic medication OFF state, because the effect of dopaminergic medication on postural control in PD appears highly heterogenous based on available evidence, making it difficult to disentangle the effects of dopaminergic treatment from contributions of other neurotransmitter systems. Nevertheless, we review available evidence on effect of dopaminergic therapy on postural control, speculate on how our findings might have looked like in the dopaminergic medication ON state, and raise questions that future studies of postural control in PD might be able to better address.
Response to anterior-posterior perturbation was reported to be improved in PD on levodopa, allowing participants with PD to more effectively counteract the force of the perturbation and remain standing instead of taking a stabilizing step backward.52 Another study showed overall greater sway ON levodopa among participants with PD, which was decreased by performance of a visual fixation task but not to the level of controls.53 During dual-task balance, levodopa both increased sway area and decreased performance on the secondary task,54 suggesting greater potential involvement of the cortex in balance on l-DOPA. Supporting this speculation is a more recent study of COP dynamics, EEG bandpower, and muscle activity following first l-DOPA administration of the day.55 COP dynamics showed greater anterior-posterior and mediolateral sway, EEG bandpower showed enhanced delta activity over motor areas, alpha and theta over parieto-occipital cortex, and beta in frontal areas, and lastly tibial anterior and gastrocnemius medialis muscle activity increased.55
Another detailed study of dynamic balance off and on levodopa characterized gastrocnemius muscle activity as a destabilizing medium latency response which is increased in the off state relative to controls, whereas tibial anterior muscle activity as stabilizing long latency response which is diminished relative to controls.56 Administration of l-DOPA fully normalized the long-latency stabilizing response, which is in agreement with improved reactive balance on l-DOPA, whereas medium-latency destabilizing response was only partially normalized.56 Delayed stabilizing long latency postural response in OFF-state is consistent with our observation of increased critical time interval, whereas increased destabilizing medium latency response in OFF-state might manifest as increased ⟨Δy2⟩c. Thus, we suspect that in ON-state, both freezers and non-freezers would probably exhibit both lower critical time interval and displacement, though differential effect of ON-state among freezers is not out of the question. Lastly, another study revealed that l-DOPA administration led to improved balance in non-fallers without dyskinesias, whereas among fallers with dyskinesias it considerably worsened sway,57 suggesting that long-term neuroplastic changes that occur in response to dopaminergic therapy must be considered when studying the effect of dopaminergic medication on postural control.
Conclusion
In conclusion, we fail to show that freezer-specific cholinergic changes related to their postural control difficulties. We were, however, able to demonstrate that freezers do experience greater postural difficulties under conditions that demand greater visual-vestibular integration. This deficit was independent of overall motor disease severity, thus reflecting freezer-specific pathological changes which we were unable to capture with our cholinergic PET analysis. Furthermore, we were able to reveal the importance of the right cortical cholinergic system for balance under visual-vestibular integration demand among both freezers and non-freezers and that its role is undermined by high anti-cholinergic burden. Our finding strengthens the case for clinical importance of managing anti-cholinergic burden among patients with PD, and that anti-cholinergic burden might be an important covariate to control for in future studies of postural control and FoG in PD. Our findings also suggest that the effect of freezer-specific cholinergic changes on postural control might be masked by compensatory processes in the relatively preserved right cerebral cortex, thus elevating the importance of accounting for the integrity of cortical cholinergic system in future analyses of subcortical cholinergic system changes related to FoG and postural instability.
Limitations
Several limitations of our findings must be acknowledged. A primary limitation of this study is the small sample size amongst freezers. Furthermore, due to the limitations of acetylcholinesterase PET we were unable to assess cholinergic integrity in most subcortical structures apart from the hippocampus, amygdala, and thalamus,14 and our lack of significant findings in those regions might still be attributable to relatively decreased sensitivity of the acetylcholinesterase PET tracer. Most importantly, the exclusion of cerebellum and striatum from our analysis due to limitations of the PET tracer leaves out possible important aspects of visual-vestibular central integration system pertaining to balance. Future studies might benefit from the use of VAChT PET to assess integrity of cholinergic system in a wider range of subcortical regions, as well as for its greater spatial specificity and ease of functional interpretation given directional differences of upregulation between these two cholinergic radioligands. Although our study was limited in the level of proof for cholinergic system changes as a shared neural underpinning for postural control deficits and FoG in advanced PD, the findings presented above advance our understanding towards eventual confirmation or falsification of this intriguing hypothesis.
Funding
This work was supported by the Department of Veterans Affairs (I01 RX001631); the Michael J. Fox Foundation; and the National Institutes of Health (grant numbers P01 NS015655, P50 NS091856, R01 NS099535, RO1 NS070856, P50 NS123067, and R01 AG073100).
Competing interests
The authors have no relevant financial or conflict of interest to disclose.
Supplementary material
Supplementary material is available at Brain online.