-
PDF
- Split View
-
Views
-
Cite
Cite
Irina V. Kulikova, Yury N. Zhuravlev, Kevin G. McCracken, Asymmetric Hybridization and Sex-Biased Gene Flow Between Eastern Spot-Billed Ducks (Anas Zonorhyncha) and Mallards (A. Platyrhynchos) in the Russian Far East, The Auk, Volume 121, Issue 3, 1 July 2004, Pages 930–949, https://doi.org/10.1093/auk/121.3.930
- Share Icon Share
Abstract
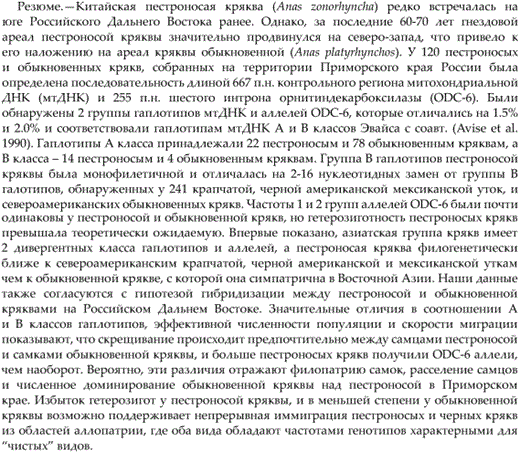
Hybridization and introgression occur in most major groups of organisms and are important components of the speciation process (e.g. Mayr 1942, 1963). For example, hybridization of formerly subdivided species or populations can provide favorable conditions for rapid evolution (Wright 1977), and reticulate evolution can substantially modify allele frequencies within and between species (Barton and Hewitt 1989, Arnold 1997, Martinsen et al. 2001, Rognon and Guyomard 2003). Among vertebrates, hybridization is common in birds (Aves). Approximately 10% of bird species are able to hybridize and produce viable offspring (Panov 1989). Waterfowl (Anatidae), in particular, are well known to produce fertile hybrids (Johnsgard 1960a, 1963; Tubaro and Lijtmaer 2002).
The ability of many different waterfowl species to hybridize raises a number of important issues about geographic structure and gene flow. For example, most waterfowl have exceptional dispersal abilities because of their capacity for flight and ability to migrate (Anderson et al. 1992), which generally are expected to promote hybridization across wide geographic areas and without simple geographic directionality. However, waterfowl also typically display strong natal fidelity, which can significantly reduce gene flow and hybridization between isolated species or populations (Rohwer and Anderson 1988, Avise et al. 1992). Sex-biased differences in dispersal and site fidelity, combined with differences in effective population size between different waterfowl species and populations, are also expected to shape patterns of hybridization and gene flow.
The Eastern Spot-billed Duck (Anas zonorhyncha) is a close relative of the Mallard (A. platyrhynchos) and one of 14 mallard species that share similar morphology, behavior, and genetics (e.g. Livezey 1991, Omland 1997, Johnson and Sorenson 1999). Eastern Spot-billed Ducks (hereafter “spot-bills”) currently occupy a breeding range in East Asia from the west coast of eastern Siberia's Lake Baikal to southern Sakhalin, including most of Japan, Korea, and northeastern China (Fig. 1). Spot-bills historically were rare in eastern Siberia and occurred infrequently in the southern Russian Far East (Polivanova 1971). During the last 60–70 years, however, the spot-bill's breeding range has expanded ∼500 km to the northwest, where they now breed in eastern Siberia (Skryabin 1963). Skryabin (1963) suggested that climate change caused expansion of the spot-bill's breeding range and brought spot-bills farther into the breeding range of the Mallard, which possesses a widespread Holarctic distribution. Breeding ranges of spot-bills and Mallards currently overlap in southeastern Siberia, the southern Russian Far East, northern Japan, and northeastern China (Fig. 1).
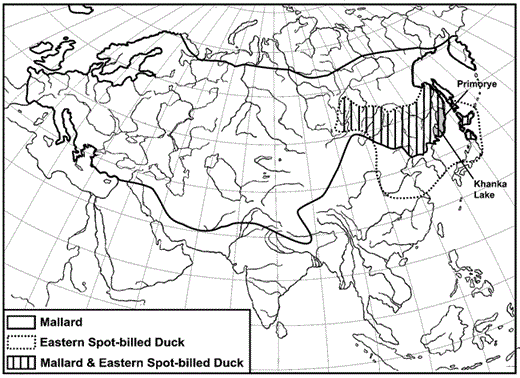
Geographic distribution of Eastern Spot-billed Ducks and Mallards in Asia.
Khanka Lake (46°N, 131°E), Primorye, is the largest freshwater lake in the Russian Far East. Approximately 1- to 2-million ducks have been recorded during spring and autumn migrations, and many thousands of ducks breed at Khanka Lake (Polivanov 1975). Early in the 20th century, spot-bills were primarily observed in the far south of Primorye and were rarely observed in other parts of the region (Polivanova 1971). However, spot-bill populations began to increase at Khanka Lake during the middle of the 20th century, though they were 6–10x less common than Mallards during the breeding season (Polivanova 1971, Gorchakov 1996). More recently, habitat at Khanka Lake has been fragmented, degraded, or eliminated because of pressure on the surrounding marshes from the local rice industry, agricultural and industrial contaminants, and wetland drainage (Bocharnikov and Glushenko 1990).
Several male A. platyrhynchos × A. zonorhyncha hybrids have recently been identified in East Asia. Melville (1999) observed four hybrids near Hong Kong, China; two hybrids were observed in winter near Tokyo, Japan (Brazil 1991, Kanouchi et al. 1998); and three hybrids were observed at Khanka Lake in Primorye during spring migration or the breeding period (Glushenko and Shibaev 1993, Shibaev and Glushenko 2001, Zhuravlev et al. 2002). Y. N. Zhuravlev (pers. obs.) recently observed several hybrids in Nagoya, Japan. The historical frequency of hybridization between spot-bills and Mallards is unknown. One hypothesis is that expansion of the spot-bill's breeding range and increased overlap of the Mallard's range has led to increased hybridization. Habitat loss because of fragmentation and wetland destruction (Bocharnikov and Glushenko 1990) may be important factors affecting hybridization as well, because with less available habitat, spot-bills and Mallards may now occur at higher densities. However, spot-bills and Mallards historically were sympatric in the southernmost regions of the Russian Far East and northern China long before the spot-bill's range expanded. Thus, hybridization may have been equally frequent historically, but undetected until recently.
Interspecific hybridization in waterfowl is well documented and common among mallard species (Johnsgard 1967, Braithwaite and Miller 1975, Rhymer et al. 1994). However, frequency of hybridization between different mallard species is difficult to measure, because many offspring of F1 × F1, or of F1 back-crossed to either parental species, typically resemble one or the other parental phenotype (Johnsgard 1967). Female F1 hybrid offspring of spot-bills and Mallards are also difficult to identify, and most spot-bill × Mallard hybrids that have been identified are males.
To investigate hybridization between spotbills and Mallards, we collected 120 spot-bills and Mallards from the Primorye region of the Russian Far East and sequenced the 5' half of the control region of the maternally inherited mitochondrial DNA (mtDNA) genome. We also sequenced 255 base pairs (bp) of the ornithine decarboxylase intron six (ODC-6; Johnson and Bulfield 1992). Our primary objectives were to (1) reconstruct the phylogenetic relationships of mtDNA haplotypes and ODC-6 alleles in spotbills and Mallards, (2) test whether frequencies of mtDNA haplotype and intron alleles differ between species, and (3) measure effective populations sizes (Ne) and migration rates (m) for mtDNA and nuclear alleles between populations of spot-bills and Mallards. To the extent possible, we also used our mtDNA and ODC-6 data to evaluate alternative hypotheses, such as ancestral polymorphism and incomplete lineage sorting, which also might account for high levels of haplotype and allele sharing and mixing in spot-bills and Mallards.
Methods
Collection, polymerase chain reaction, and DNA sequencing
We obtained liver tissue from 28 spotbills and 83 Mallards collected during the 1999-2002 hunting seasons in Primorye, Russia (Table 1). We collected wing feathers from seven spot-bill museum skins and two spot-bill × Mallard phenotypic hybrids that possessed intermediate feather and bill colors (Glushenko and Shibaev 1993, Zhuravlev et al. 2002).
Sources of genetic material and numbers of Eastern Spot-billed Ducks and Mallards collected from Primorye, Russia.
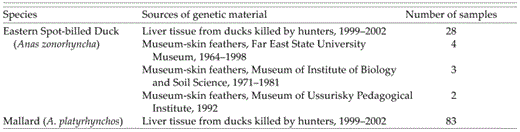
Sources of genetic material and numbers of Eastern Spot-billed Ducks and Mallards collected from Primorye, Russia.
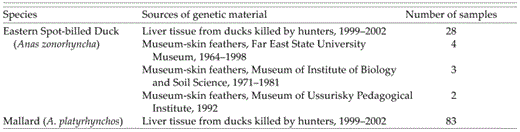
We extracted DNA from tissue or the base of one or two feather quills using a DNeasy Tissue Kit (Qiagen, Valencia, California). We added 30 μL of 100 mg mL−1 dithiothreitol to the digestion buffer to dissolve feather quills. We used polymerase chain reaction (PCR) to amplify the 5' half of the mtDNA control region (positions 79–773 in the chicken [Gallus gallus] mtDNA; Desjardins and Morais 1990). Controlregion primers included Sorenson and Fleischer's (1996) L78 and Sorenson et al.'s (1999) H774. We used L78 and H493 (Sorenson and Fleischer 1996) for most of the feather samples, but used L78 and H219 (5'-GTTGGTRGKRTATGTCTRTCAAGC-3') for one spot-bill feather sample, because amplifications of the L78-H774 or L78-H493 fragments were not successful. We used ODC-6 primers (ODC-6.F 5′GCTGCTTCTGGAGCGTGCAAG-3′; ODC-7.R 5′CTCCCATATCAAACACACAGCGG-3′) to amplify 255 bp; primers were designed by M. D. Sorenson, based on sequences for chicken and other species downloaded from GenBank.
The PCR reactions were carried out in a DNA Engine DYAD (MJ Research, Waltham, Massachusetts) thermal cycler using 50 μL reactions, containing 2 μL template DNA, 2.5 μL of each primer (10 μm), 5 μL of 10 μm dNTPs, 5 μL of 25 mM MgCl2, 5 μL of 10× PCR buffer, and 0.25 μL Taq Polymerase. We used AmpliTaq Gold PCR Master Mix (Applied Biosystems, Foster City, California) for the feather samples and introns. Thermal cycling for mtDNA was as follows: 7 min preheat at 94°C, followed by 45 cycles of 20 s at 94°C, 20 s at 52°C, 1 min at 72°C, and a final extension of 7 min at 72°C. We used a 64°C annealing temperature (instead of 52°C) for the ODC-6 introns.
The PCR products were electrophoresed in agarose, excised from the gel, and purified using QIAquick Gel Extraction Kits (Qiagen, Valencia, California). Both strands of each control region product were cyclesequenced in 10-μL reactions using BigDye Terminator Cycle Sequencing Kits diluted 4-fold, followed by electrophoresis on an ABI 3100 automated DNA-sequencer (Applied Biosystems, Foster City, California). Sequences from opposite mtDNA strands were reconciled and verified for accuracy using SEQUENCHER 4.1 (Gene Codes, Ann Arbor, Michigan). For the ODC-6 introns, we sequenced only the forward strand because the PCR template was short (255 bp) and because AmpliTaq Gold and a 64°C annealing temperature produced consistent peak height throughout the length of the sequence. Nonetheless, we randomly selected and resequenced 10% of individuals to verify results. Double-peaks in ODC-6 reflecting the presence of two different alleles were evaluated for all polymorphic positions by carefully comparing each putatively heterozygous sequence to each other sequence in the data set, including those from the subset of individuals that were sequenced twice. Double-peaks subsequently were coded with IUPAC degeneracy codes and treated as polymorphisms. Sequences are archived in GenBank (accession numbers AY506868–AY507105).
Control-region data analysis
We used PAUP* 4.0b10 (Swofford 1998) and unweighted parsimony to estimate phylogenetic relationships of unique control-region haplotypes observed in spot-bills and Mallards from Primorye. We included 57 unique haplotypes from Mottled (A. fulvigula; n = 40), American Black (A. rubripes; n = 10), and Mexican (A. diazi; n = 1) ducks, and Mallards (n = 6), all from North America, in the analysis (McCracken et al. 2001). Subsequent analyses included only spot-bills and Mallards from Primorye (see below). Tree searches were performed in two steps: 500 random-addition replicates, each limited to 100 trees, followed by a single search, with no limit, using all trees from the first round as starting trees. Searches were heuristic, with TBR (tree bisection and reconnection) branch swapping and gaps coded as a fifth-character state. Two divergent clades of haplotypes shared by spotbills and Mallards were observed, and all trees were rooted on the mid-point. We used TCS (Clement et al. 2000) to illustrate unrooted networks for each clade of haplotypes.
We measured number of unique haplotypes and percentage of variable nucleotide sites for each species; we estimated net nucleotide divergence between the two haplotype clades, using MEGA 2.0 (Kumar et al. 2001). For each species, we calculated haplotype diversity (h) and nucleotide diversity (π) (Nei 1987) using ARLEQUIN 2.0 (Schneider et al. 2000). For those calculations, genetic distances were corrected for multiple hits by the method of Kimura (1980) assuming a gamma-shape parameter (α = 0.90) determined in MODELTEST 3.06 (Posada and Crandall 1998). We performed analysis of molecular variance (AMOVA; Excoffier et al. 1992) and calculated population pairwise FST test statistics and corresponding P-values between spot-bills and Mallards based on the AMOVA model implemented in ARLEQUIN (Schneider et al. 2000). Pairwise mismatch distributions (e.g. Slatkin and Hudson 1991) and Rogers' (1995) model of sudden population expansion for species and haplotype lineages were also calculated using ARLEQUIN (Schneider et al. 2000).
Ornithine decarboxylase intron-six data analysis
We estimated the phylogenetic relationships of 54 ODC6 allele sequences from 27 spot-bills (n = 2) and Mallards (n = 25), which were either homozygotes (n = 8) or heterozygotes (n = 19) that possessed one polymorphic position. Thus, the gametic phase of each of those 27 individuals was unambiguous; we identified 11 different ODC-6 alleles in that manner. The ODC-6 allele sequences for the remaining 93 individuals were all heterozygotes that possessed two or more polymorphic nucleotide positions, and those were omitted from the next step of the analysis. We used the PAUP* 4.0b10 (Swofford 1998) branch-andbound-search algorithm and statistical parsimony implemented in TCS (Clement et al. 2000) to illustrate the 95% set of unrooted allele trees for the 54 unambiguous alleles. Two divergent clades of allele sequences (e.g. types 1 and 2) were observed for the ODC-6 locus, and all trees were rooted on the mid-point. We reconstructed ancestral character-state changes for each variable nucleotide position, using unweighted parsimony; by doing so, we identified five nucleotide positions that showed fixed differences between the type 1 and 2 clades. All 120 individuals in our ODC-6 data set (including the remaining 93 heterozygotes) were subsequently scored for those polymorphic positions and determined to be one of three genotypes: (1) type 1:1, (2) type 1:2, or (3) type 2:2 (see below). We used those genotypic data and ARLEQUIN (Schneider et al. 2000) to calculate pairwise FST for spot-bills and Mallards and used the chi-square distribution to test whether ODC-6 genotypic frequencies of spot-bills and Mallards were in Hardy-Weinberg equilibrium.
Effective population size and migration rates
We used maximum-likelihood (ML) and coalescent analysis implemented in MIGRATE 1.6.9 (Beerli and Felsenstein 1999) to estimate Ne and m, on the basis of the neutral parameter theta (θ), which is equivalent to Neμ for the maternally inherited mtDNA control region and 4Neμ for the biparentally inherited ODC-6 locus. We used a source-sink model (Mallard → spot-bill) for the control region, because (1) spot-bill and Mallard haplotype frequencies show evidence of asymmetric gene flow, (2) Mallards outnumber spot-bills in Primorye, and (3) the same two divergent groups of haplotypes occur in both species but at very different frequencies (Table 2; Beerli and Felsenstein 2001). For the ODC-6 locus, which showed approximately equal frequencies of type 1 and 2 alleles in both species, we used the source-sink model (Mallard → spot-bill) and a two-island model (spot-bill ↔ Mallard), which does not assume a priori directionality or asymmetry to gene flow. A DNA sequence model with empirical base frequencies and a transition bias of 31.69 was used for the mtDNA data, whereas an allelic model (genotype = 11, 12, or 22) was used for the ODC-6 locus. Start values for θ and m were estimated using FST, assuming variable m and constant θ.
Group A and B mtDNA control-region haplotype frequencies and ornithine decarboxylase intron six (ODC-6) allele and genotype frequencies, including observed and expected frequencies, for Eastern Spot-billed Ducks and Mallards from Primorye, Russia. Samples sizes for the control region were 36 spot-bills and 82 Mallards, whereas samples sizes for ODC-6 were 37 spot-bills and 83 Mallards (the two phenotypic hybrids were classified as spot-bills).
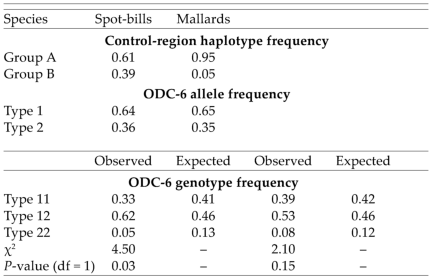
Group A and B mtDNA control-region haplotype frequencies and ornithine decarboxylase intron six (ODC-6) allele and genotype frequencies, including observed and expected frequencies, for Eastern Spot-billed Ducks and Mallards from Primorye, Russia. Samples sizes for the control region were 36 spot-bills and 82 Mallards, whereas samples sizes for ODC-6 were 37 spot-bills and 83 Mallards (the two phenotypic hybrids were classified as spot-bills).
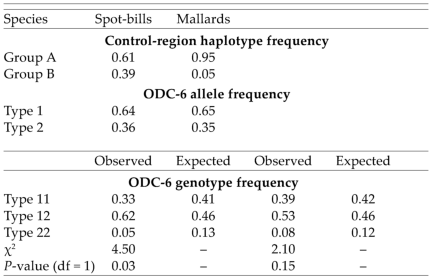
Results
Maternally inherited mitochondrial DNA control region
We identified 79 unique haplotypes composed of 666–667 nucleotides in 118 controlregion sequences, or approximately two for every three individuals sequenced. One nucleotide deletion at position 211 was observed in 15 spot-bills and in 7 Mallards (see McCracken et al. 2001), and single nucleotide deletions at positions 206 and 210 were observed in one spotbill and in one Mallard, respectively. Of the 667 positions, 68 (10.2%) varied among haplotypes. Transitions occurred at 66 positions (97.1%), and transversions occurred at 4 positions (5.9%). All but one substitution occurred within the first 351 positions relative to the 5' end of the 667 bp sequenced; the other substitution occurred at position 521. Nucleotide composition was 33.4% C, 25.7% T, 25.6% A, and 15.3% G. Average percentage of GC content (48.7%) was similar to that of other birds (e.g. Baker and Marshall 1997, Randi and Lucchini 1998). No ambiguous or coamplified nucleotides were observed.
Fifty-seven haplotypes (72.1%) occurred in only one individual, 19 haplotypes (24.1%) were shared by 2–3 individuals, and 3 haplotypes (3.8%) were shared by 5–8 individuals. Haplotype diversity (h ± V[h]) was 0.9619 ± 0.0187 for spot-bills and 0.9868 ± 0.0061 for Mallards. Nucleotide diversity (π ± V[π]) was 0.01369 ± 0.0072 for spot-bills and 0.0083 ± 0.0045 for Mallards. Fifty-six haplotypes (70.1%) occurred only in Mallards, 18 (22.8%) occurred only in spot-bills, and 5 (6.3%) occurred in spotbills and Mallards.
Unweighted parsimony analysis of 9 spot-bill and Mallard haplotypes from Primorye and 57 Mottled Duck, American Black Duck, Mexican Duck, and Mallard haplotypes from North America (McCracken et al. 2001) produced hundreds of thousands of most-parsimonious (MP) trees (length = 199, consistency index [CI] = 0.47, retention index [RI] = 0.90). Strict consensus shows two divergent haplotype groups separated by 10–30 substitutions, which correspond to Avise et al.'s (1990) group A and B haplotypes and type 1 and type 2 haplotypes identified by Johnson and Sorenson (1999) (see also McCracken et al. 2001). Haplotype relationships for one randomly chosen MP tree rooted on the mid-point and unrooted haplotype networks for each species are illustrated in Figures 2 and 3. The group A haplotype clade is composed of spot-bills and Mallards from Asia and North America; whereas the group B haplotype clade is composed of spot-bills; Mallards; and Mottled, American Black, and Mexican ducks from Asia and North America. Spot-bills and Mallards from southeastern Russia share two distinct haplotype lineages (groups A and B), but the frequency of group A and B haplotypes differs between species and between Asia and North America (Table 2; Fig. 3).
(A) Unrooted parsimony tree illustrating phylogenetic relationships of group A mtDNA control-region haplotypes. Haplotype numbers correspond to numbers in Figure 2; sizes of ellipses are proportional to number of individuals that possess the haplotype. Small ellipses indicate intermediate ancestral haplotypes that were not sampled. Figure 3 is continued on the next page.
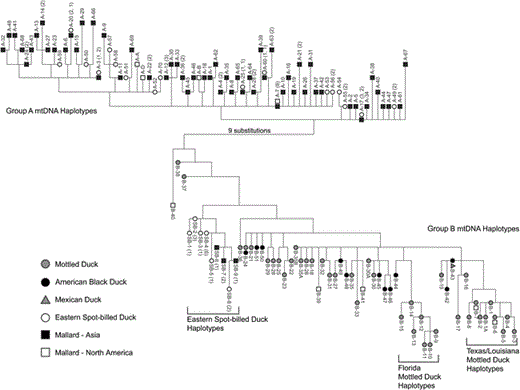
Most-parsimonious tree illustrating phylogenetic relationships of group A and B mtDNA controlregion haplotypes found in Eastern Spot-billed Ducks; Mallards; and Mottled, American Black, and Mexican ducks (length = 199, CI = 0.47, RI = 0.90). Group A and B haplotypes shared by spot-bills and Mallards from Primorye are labeled with number of spot-bills and Mallards that shared the haplotype in parentheses (group A: no. Mallards, no. spot-bills; group B: no. spot-bills or no. Mallards). Group B haplotype numbers (B1, B2, etc.) for individuals sampled from North America correspond to haplotype numbers listed in Genbank by McCracken et al. (2001), whereas group A (e.g. A-1, A-2) and B spot-bill (e.g. SB-1) haplotype numbers correspond to the present study.
Group A haplotypes occurred in 22 spotbills, 78 Mallards from Asia, and 5 Mallards from North America. No obvious phylogenetic structure was apparent within that clade; most group A haplotypes differed from other group A haplotypes by a single substitution (Figs. 2 and 3). In contrast, group B haplotypes possessed three distinct clades: (1) a clade comprising 14 spot-bills (6 haplotypes) and 4 Asian Mallards (3 haplotypes); (2) a clade composed exclusively of Florida Mot-tled Ducks (7 haplotypes); and (3) a clade comprising Texas and Louisiana Mottled Ducks and North American Mallards (9 haplotypes). All other group B haplotypes occurred in Mottled, American Black, or Mexican ducks, or in Mallards from North America. Asian Mallards with group B haplotypes occurred only in the clade of haplotypes shared with spot-bills. Finally, one spot-bill × Mallard hybrid possessed a group A haplotype; the other possessed a group B haplotype.
In summary, parsimony analysis indicates that group A and B haplotypes observed in North America by Avise et al. (1990), Johnson and Sorenson (1999), and McCracken et al. (2001) also occur in Asia. Group A haplotypes were observed exclusively within spot-bills and Mallards; whereas group B haplotypes were observed in spot-bills; Mallards; and Mottled, American Black, and Mexican ducks. A previously undescribed subclade of group B haplotypes (group SB) was also observed, and 14 individuals possessing those haplotypes were spot-bills; the other 4 individuals were Asian Mallards. No group SB haplotypes have been identified in mallard species from North America.
Fixation index for spot-bills and Asian Mallards was significant (FST = 0.19, P < 0.0001). Analysis of molecular variance indicated that 80.7% of genetic variation occurred within species, whereas 19.3% occurred between species. Analysis of molecular variance indicated that 78.7% of genetic variation occurred between group A and B lineages, and 21.3% occurred within lineages.
Mismatch distributions for group A and B haplotypes were distributed normally and did not differ from Rogers' (1995) model of sudden population expansion following a recent bottleneck (Ps > 0.13; Fig. 4A, B). In contrast, pairwise mismatch distributions for spot-bills and Mallards were distributed multimodally and bimodally, respectively, and differed from Rogers' (1995) model (Ps < 0.02; Fig. 4C, D).
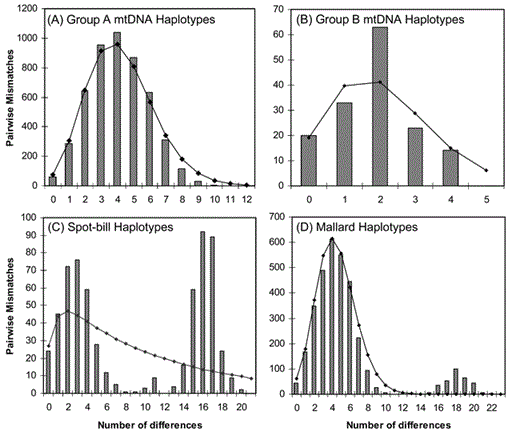
Pairwise mismatch distributions for (A) group A mtDNA control-region haplotypes; (B) group B mtDNA control-region haplotypes; (C) Eastern Spot-bill Duck control-region mtDNA haplotypes; and (D) Mallard control-region mtDNA haplotypes. Expected number of pairwise mismatches from Rogers' (1995) model of sudden population expansion are indicated by black line in each panel.
Biparentally inherited ornithine decarboxylase intron-six
We identified 23 polymorphic nucleotide positions in the ODC-6 allele sequences of 120 spot-bills and Mallards. Thirteen positions (56.5%) segregated for transitions, 9 positions (39.1%) segregated for transversions, and 1 position (4.4%) segregated for transitions and transversions. Eight individuals (6.7%; 1 spot-bill, 7 Mallards) were homozygotes, and 19 individuals (15.8%; 1 spot-bill, 18 Mallards) were heterozygotes that possessed one polymorphic position. The gametic phases of 54 allele sequences for those 27 individuals were unambiguous and included 11 unique ODC-6 allele sequences. Unweighted parsimony analysis of the 11 allele sequences yielded 14 most- parsimonious trees (length = 15, CI = 0.87, RI = 0.91). The 95% confidence set of unrooted allele trees for the 27 spot-bills and Mallards is illustrated in Figure 5. Two clades of alleles that differ by fixed nucleotide differences at five positions (2.0%) are evident in the tree. Type 1 alleles are fixed for C, C, G, C, and T at positions 156, 160, 162, 167, and 177, respectively; whereas type 2 alleles are fixed for A, T, C, T, and C (Fig. 5). Each of the remaining individuals in our data set were heterozygotes with two or more polymorphic positions. All those individuals showed one of only three different allele sequences at positions 156, 160, 162, 167, and 177: CCGCT, MYSYY, or ATCTC.
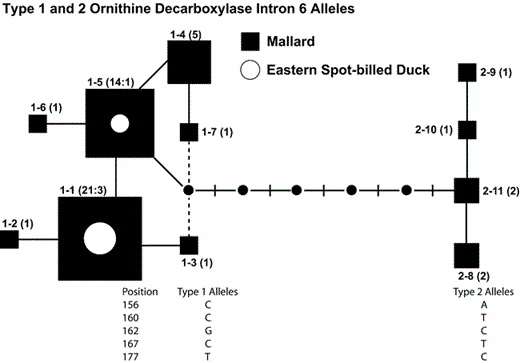
One of 14 most-parsimonious trees (length = 15, CI = 0.87. RI = 0.91), illustrating phylogenetic relationships of 11 ornithine decarboxylase intron six (ODC-6) alleles identified in spot-bills and Mallards that were either homozygotes or polymorphic at one nucleotide position. Numbers in parentheses and size of each allele are proportional to number of spot-bills (circles) and Mallards (squares) from Primorye that possess the allele. Dashed lines connecting alleles show alternative branches included in the 95% set of unrooted parsimony trees. Nucleotides occurring at positions 156, 160, 162, 167, and 177 in type 1 and 2 allele groups are listed below the tree. Small black circles indicate intermediate ancestral alleles that were not sampled.
On the basis of that finding, we scored the allelic phases of all individuals (including all heterozygotes) as type 11 (CCGCT), type 12 (MYSYY), or type 22 (ATCTC) and calculated allelic and genotypic frequencies. Allelic frequencies were approximately equal in spot-bills and Mallards, and the genotypic frequencies that we observed did not differ between species (FST = 0.0, P = 0.89; Table 2). However, spot-bills possessed more heterozygotes and fewer homozygotes than expected under Hardy-Weinberg equilibrium conditions (χ2 > 4.50, df = 1, P = 0.03; Table 2). Mallards also possessed excess heterozygotes, but genotypic frequencies did not differ from Hardy-Weinberg proportions (χ2 > 2.10, df = 1, P = 0.15).
Effective population size and migration rates
Maximum-likelihood estimates of theta (Neμ, 4Neμ) and migration rates (Nem, 4Nem) for source-sink and two-island models of population subdivision were determined for mtDNA and ODC-6 (Table 3). The 95% confidence intervals (CI) indicate that theta, and thus Ne (assuming a constant mutation rate), was smaller for spotbills than Mallards for both loci. For source-sink models, number of migrant Mallard mtDNA haplotypes that entered the spot-bill population was 12.89 (95% CI; 7.43–17.98) per generation; number of ODC-6 alleles that entered the spot-bill population was 0.11 (95% CI; 0.01–1.28) per generation. The two-island model for ODC-6 indicated that number of Mallard alleles that entered the spotbill population was 4.41 (95% CI; 0.22–7.67) per generation; number of spot-bill alleles that entered the Mallard population was <<0.01 (95% CI; 0.00– 0.52) per generation. Applying a two-island model of population subdivision to the mtDNA control region resulted in an estimate of a small migration rate (Mallard → spot-bill; 95% CI = 0.11–3.69) in the wrong direction, and an erroneously large estimate in the opposite direction (spot-bill → Mallard; 95% CI = 31.4–115.14). Reversing the source-sink model (spot-bill → Mallard), likewise, resulted in an erroneous migration rate (95% CI = 59.56–143.49). Those estimates are incorrect because many more spot-bills (61%) received group A mtDNA haplotypes than Mallards (5%) received group B haplotype (Table 2). They also reflect the limits of ML estimation that can occur when one or more haplotypes shared by two different populations occur at very low frequency in one of the populations (Beerli and Felsenstein 2001).
Neutral parameters (θ) and migration rates (m) for mtDNA control-region haplotypes and ornithine decarboxylase intron-six (ODC-6) alleles of Eastern Spot-billed Ducks (“spot-bills”) and Mallards from Primorye, Russia.
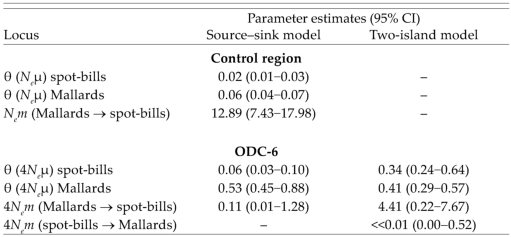
Neutral parameters (θ) and migration rates (m) for mtDNA control-region haplotypes and ornithine decarboxylase intron-six (ODC-6) alleles of Eastern Spot-billed Ducks (“spot-bills”) and Mallards from Primorye, Russia.
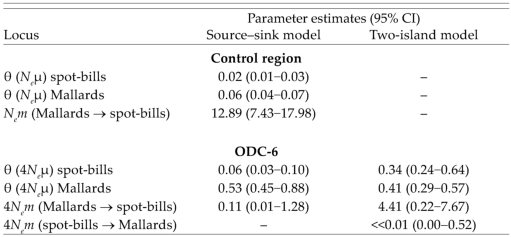
Discussion
Historical phylogeography
The nominate Mallard is the only mallard species that is strongly sexually dichromatic and possesses a Holarctic distribution. The other 13 mallard species are predominantly monochromatic and possess more restricted geographic ranges (Omland 1997, McCracken et al. 2001). In addition, Mallards routinely hybridize with other mallard species—for example, Pacific Black Duck (A. superciliosa) in Australia (Braithwaite and Miller 1975) and New Zealand (Gillespie 1985, Rhymer et al. 1994); Mottled, American Black, and Mexican ducks in North America (Johnsgard 1967, Mazourek and Gray 1994); and Hawaiian Duck (A. wyvilliana) in the Hawaiian Islands (Griffin et al. 1989).
Ankney et al. (1986) and Avise et al. (1990) first studied genetic differentiation between Mallards and Black Ducks using allozymes and restriction-enzyme-digested mtDNA. Two mtDNA haplotype groups were observed in North America: a group A lineage comprising Mallards and a group B lineage comprising Mallards and black ducks. Avise et al. (1990) proposed incomplete matriarchal lineage sorting from a polymorphic ancestral gene pool as the explanation for paraphyly of Mallard and black duck haplotypes. Since then, Mallard haplotypes have also been shown to be paraphyletic with mtDNA haplotypes of Hawaiian Duck (Cooper et al. 1996, Rhymer 2001); and Mottled, American Black, and Mexican ducks (Johnson and Sorenson 1999, McCracken et al. 2001). McCracken et al. (2001) also demonstrated that Mottled Ducks primarily possess one group of mtDNA haplotype (group B), whereas North American Mallards sampled in the same study possessed two groups of mtDNA haplotypes (groups A and B). In contrast, Mallards from Europe and Asia that were assayed previously possessed the group A haplotype group (Young and Rhymer 1998), similarly found by Johnson and Sorenson for (1999) spot-bills, Spot-billed Ducks (A. poecilorhyncha), and Phillipine Ducks (A. luzonica).
Paraphyly of mallard species mtDNA haplotypes and hybridization between Mallards and their close relatives have been widely discussed in the literature (e.g. Johnsgard 1961, 1965; Ankney et al. 1986; Ankney and Dennis 1988; Hepp et al. 1988; Avise et al. 1990; Rhymer et al. 1994; Cooper et al. 1996; Omland 1997; Johnson and Sorenson 1999; McCracken et al. 2001; Rhymer 2001). Omland (1997) argued that a dichromatic Mallard-like common ancestor gave rise to the various monochromatic mallard species by peripatric speciation and, like Avise et al. (1990), concluded that sharing and mixing of group A and B mtDNA haplotypes in New World mallard species was the result of ancestral polymorphism and incomplete lineage sorting. In contrast, Palmer (1976), Johnson and Sorenson (1999), and McCracken et al (2001) proposed that nominate Mallard originated in the Old World, probably in Asia, subsequently colonized the New World, and in the process acquired American Black, Mottled, and Mexican duck mtDNA by hybridization with those monochromatic mallard species, which colonized North America prior to nominate Mallards. According to that viewpoint, sharing and mixing of group A and B mtDNA haplotypes have resulted from hybridization and widespread introgression.
Our analysis is the first to identify two divergent haplotype and allele lineages (group A and B, type 1 and 2) in Asian mallard species. Parsimony analysis clearly reveals two divergent clades of haplotypes and alleles in Asia, and each clade contains spot-bills and Mallards. Pairwise mismatch distributions for both species mtDNA also possess two or more major peaks: (1) those showing low to moderate numbers of nucleotide differences, and (2) those showing large numbers of differences. Those patterns reflect the existence of deeply divergent mtDNA lineages in Asian spot-bills and Mallards. Fixation index also shows low levels of fixation between species (mtDNA FST = 0.19; ODC-6 FST = 0.0). In addition, identity by descent of group A and B mtDNA haplotypes found in Asia and North America is supported by combined analysis of Mottled, American Black, and Mexican ducks, and Mallards from North America.
Moreover, the mismatch distribution and starlike pattern of the group A mtDNA haplotype group suggests that species carrying group A haplotypes experienced a recent population expansion. The mismatch distribution for the group B haplotypes does not rule out Rogers' (1995) model of sudden population expansion, but fits the model less well than the group A haplotypes. Those results, and patterns and branch lengths, suggest that the group A haplotypes are more recently diverged, but that the group B haplotypes possess a deeper evolutionary history. That hypothesis is confirmed by the fact that the group B mtDNA haplotype group possesses three distinct clades: (1) a clade comprising primarily spot-bills, (2) a clade comprising exclusively of Florida Mottled Ducks, and (3) a clade comprising primarily Texas and Louisiana Mottled Ducks (see McCracken et al. 2001). No such clade structure is apparent for group A haplotypes.
Net nucleotide divergence (±SE) between group A and B mtDNA haplotypes is 3.8% ± 1.1%. Using a clock calibration of 8.8% sequence evolution per million years for 5′-end of the duck mitochondrial control region (Sorenson and Fleischer 1996), group A and B haplotype lineages are estimated to have diverged in the Pleistocene, 430,000 years ago (95% CI = 180,000–680,000 years ago). That estimate is equal to the number Avise et al. (1990) obtained from restriction-site mtDNA data and suggests that the group A and B haplotypes diverged in allopatry hundreds of thousands of years ago.
Hybridization or ancestral polymorphism?
The basis of the hybridization argument is that group A and B mtDNA haplotypes and type 1 and 2 ODC-6 allele diverged in allopatry, and previously isolated mallard populations experienced gene flow because of recent and continuing introgression. If divergent haplotypes and alleles resulted from ancestral polymorphism and incomplete lineage sorting without hybridization, paraphyly reflects a very old—and lasting—discordance between gene and species trees (e.g. Avise 2000) that is widespread over different continents and many species.
In general, the probability that a gene and species tree will conflict because of lineage sorting and retention of polymorphisms across adjacent nodes is large when 2Ne > T, where T is duration of the internode between deep coalescence and population divergence (Nei 1987, Avise 2000). Nominate Mallards are widespread, have experienced recent population growth, have large Ne and, thus, are good candidates to study for persistent effects of old ancestral polymorphism. Nonetheless, spot-bills and Mallards might be expected to possess divergent haplotype and allele sequences within each A-B gene lineage, provided that the two populations diverged sufficiently long ago for diagnostic differences to accumulate. However, that pattern was not observed; homotypic mtDNA haplotypes and alleles of spot-bills and Mallards are identical or nearly identical (1–2 bp). Nonetheless, successive periods of rapid population growth throughout the period of lineage sorting are likewise expected to increase the probability of paraphyly and a mismatch between gene and species trees. Yet another possibility that must be evaluated is that paraphyly originated very early, as a consequence of past hybridization between older ancestors, but that hybridization subsequently ceased. That alternative is seemingly inconsistent with empirical data, because ancient hybridization followed by population subdivision would also predict that extant spot-bills and Mallards should show deeply diverged haplotypes and alleles within the same homotypic lineages.
Can mtDNA and nuclear genotypic data from spot-bills and Mallards from the Russian Far East shed light on the debate about the relative importance of hybridization and ancestral polymorphism? We believe that there are five important lines of evidence that support hybridization as a more consistent explanation for sharing and mixing of group A and B haplotypes and type 1 and 2 alleles in spot-bills and Mallards than ancestral polymorphism and incomplete lineage sorting.
(1) Hybridization between spot-bills and Mallards is well documented (Brazil 1991, Glushenko and Shibaev 1993, Kanouchi et al. 1998, Melville 1999, Shibaev and Glushenko 2001, Zhuravlev et al. 2002), and similar patterns of hybridization are common for many other mallard species (e.g. Johnsgard 1967; Braithwaite and Miller 1975; Rhymer et al. 1994, 2001). The debate is not about whether hybridization frequently occurs per se, but whether hybridization is principally responsible for patterns of group A and B mtDNA haplotype and type 1 and 2 allele sharing and mixing.
(2) Two divergent allele types (i.e. type 1 or 2) have been identified for ODC-6, beta fibrinogen intron 7 (BF-7), and a variety of other nuclear loci (I. V. Kulikova et al. unpubl. data; M. D. Sorenson and R. J. Harrigan pers. comm.). Consistent patterns of divergence for multiple unlinked loci might reflect a shared history of vicariance, allopatry, and divergence, instead of stochastic processes such as ancestral polymorphism and incomplete lineage sorting. Identifying which allele groups are associated with the group A or B mtDNA haplotype is problematic and warrants future inquiry. Determining which allele types are correlated with organismal and geographical barriers ultimately may resolve debate.
(3) Control-region data from 39 additional Mallards collected in areas of allopatry outside the Primorye region, where spot-bills do not routinely occur, indicate that Mallards in those regions are fixed for the group A mtDNA haplotype (e.g. elsewhere in East Asia and Europe; n = 22) or show low frequencies (<12%) of the group B haplotype (e.g. Aleutian Islands, n = 17; I. V. Kulikova et al. unpubl. data). Moreover, the only group B mtDNA haplotypes identified among 104 Old World Mallards (excluding Mallards from the Aleutian Islands) are group SB haplotypes, which, to date, have been observed only in spot-bills or Mallards from Primorye. That geographic structure is consistent with allopatric speciation followed by hybridization, but it is inconsistent with ancestral polymorphism and incomplete lineage sorting, because the latter are expected to result in the random distribution of each haplotype and allele group in different geographic areas.
(4) Spot-bills and Mallards show excess heterozygosity at the ODC-6 locus in Primorye, and spot-bill heterozygosity is greater than expected under Hardy-Weinberg equilibrium. Excess heterozygosity is a common and expected result of hybridization between populations (e.g. spot-bills and Mallards) that possess different allele frequencies in adjacent regions of allopatry. In contrast, ancestral polymorphism and incomplete lineage sorting are not expected to cause excess heterozygosity or departure from Hardy-Weinberg equilibrium, because equilibrium should be attained after one generation of random mating, regardless of initial allele frequencies.
(5) Plumage similarities between spot-bills and American Black, Mottled, and Mexican ducks are consistent with the hypothesis that hybridization is the principal cause of shared haplotypes and alleles (see Palmer 1976, McCracken et al. 2001). Among the world's 14 mallard species, nominate Mallard is the only species that is strongly sexually dichromatic. Reconstructing dichromatic plumage as the ancestral plumage state for mallard species (albeit consistent with ancestral polymorphism and incomplete lineage sorting; e.g. Omland 1997) requires the potentially illjustified assumption that loss of dichromatism is at least five times more likely than gain.
We believe that those arguments favor hybridization as the primary cause of sharing and mixing of divergent haplotypes and alleles in spot-bills and Mallards. In a broader context, those arguments are also consistent with the “Asian invasion” hypothesis first articulated by Palmer (1976) and Johnson and Sorenson (1999). Nonetheless, the alternative hypothesis of ancestral polymorphism and incomplete lineage sorting cannot be ruled out until haplotype and allele frequencies are obtained for spot-bills and additional Mallards from areas of allopatry where Mallards do not routinely occur (i.e. southeast Asia) and genotypes of other closely related New World and Old World mallard species are examined.
Eastern Spot-billed Duck × Mallard hybridization
If group A and B mtDNA haplotypes and type 1 and type 2 ODC-6 alleles diverged in allopatry, and haplotype and allele sharing and mixing are the result of hybridization, it is useful to ask: Which mtDNA haplotype and ODC-6 allele groups were characteristic historically of spot-bill and Mallard ancestors? The question presupposes a typological DNA species concept but may be instructive, nonetheless, if the basic premises are correct.
Johnson and Sorenson (1999) and McCracken et al. (2001) proposed that group A mtDNA haplotypes originally belonged to ancestral Mallards, but until now, no data were available for Asian Mallards or spot-bills. Group A mtDNA haplotypes occurred in 22 spot-bills and 78 Mallards from Primorye, and group B haplotypes occurred in 14 spot-bills and 4 Mallards. Our data further indicate that the clade of group B haplotypes that occurred in spot-bills (group SB) is monophyletic and diverged by 3–11 substitutions from the group B haplotypes that occurred in Mottled, American Black, and Mexican ducks, and in North American Mallards. Thus, group B haplotypes occur in Asia, but the spot-bills and Asian Mallards that we sampled possess only a subset of group B (group SB) haplotypes, which are yet to be identified in the New World. In contrast to the spot-bills sequenced by Johnson and Sorenson (1999), which possessed a group A haplotype, those findings suggest that spot-bills are not close relatives of Asian Mallards but instead are more closely related to Mottled, American Black, and Mexican ducks. That hypothesis agrees with plumage morphology. Spot-bill plumages are more similar to the plumage of Mottled, American Black, or Mexican ducks than to Mallard plumage. One possible explanation for the discrepancy between the two studies is that Johnson and Sorenson (1999) sequenced a captive spot-bill that acquired a Mallard haplotype via hybridization either naturally or in an aviary. In summary, we agree with Johnson and Sorenson (1999) and McCracken et al. (2001) and further conclude that the group A haplotype group might be the ancestral Mallard haplotype.
Determining which ODC-6 allele sequence (type 1 or 2) historically diverged in the ancestors of Mallards and which diverged in the ancestors of spot-bills is more problematic. If ancestral polymorphism and incomplete lineage sorting are the root causes of haplotype and allele sharing and mixing, no correspondence between group A and B mtDNA haplotypes and type 1 and 2 ODC-6 alleles would be expected. However, if the haplotype and allele groups diverged in allopatry in response to the same vicariance event, correspondence between group A and B mtDNA haplotypes, type 1 and 2 ODC-6 alleles, and similarly divergent allele groups at additional loci (e.g. BF-7, etc.) could be expected. Spot-bills and Mallards from Primorye show equal frequencies of type 1 and 2 ODC-6 allele sequences, and the type 1 allele is much more common (64–65%) than the type 2 allele (35–36%). On the basis of patterns of asymmetry in hybridization and apparent differences in Ne (see below), we hypothesize that the more common type 1 ODC-6 allele was historically characteristic of Mallards and that the type 2 ODC-6 allele sequence historically belonged to spot-bills prior to widespread hybridization, but that hypothesis warrants further testing. Finally, the five polymorphisms that we used to score those alleles span 22 bp, and effects of recombination were not evident on those five positions.
Asymmetric hybridization
Bias in the group A:B mtDNA haplotype ratios in spot-bills (1.6:1) and Mallards (19.5:1) suggests that hybridization between the two species has occurred asymmetrically (i.e. many more spot-bills received Mallard mtDNA than vice versa). Because mtDNA is maternally inherited, that means that male spot-bills mated with female Mallards in much greater numbers (Nem = 12.89; 95% CI = 7.43–17.98; Tables 2 and 3) than female spot-bills mated with male Mallards. It is unfortunate that Nem could not be calculated for mtDNA gene flow arising from female spotbills that mated with male Mallards because of limits of the ML estimation procedure (Beerli and Felsenstein 2001). However, low frequencies of group B haplotypes in the Mallard population that we sampled (5%) support the hypothesis that asymmetric migration of mtDNA haplotypes occurred in those species, as do estimates of 4Nem for the ODC-6 locus using a two-island model of population subdivision. If the ODC-6 type 2 allele was historically characteristic of spot-bills (as we predict), asymmetric migration must also have occurred historically at the ODC-6 locus, because twothirds of the spot-bill alleles we sampled are type 1 alleles. In fact, both species possess two-thirds of type 1 alleles and one-third of type 2 alleles, which might reflect differences in Ne that we observed between species and the source—sink dynamics that have occurred in Primorye. Finally, spot-bills show increased heterozygosity and are not in Hardy-Weinberg equilibrium at the ODC-6 locus, which is expected if the historically allopatric spot-bill genome has become strongly admixed because of hybridization with Mallards.
Some authors have suggested that hybridization between mallard species frequently occurs asymmetrically, because dichromatic male Mallards are dominant to nondichromatic males or because females of the nondichromatic species are preferentially attracted to the colorful plumage of the male Mallard (Johnsgard 1960b, Brodsky and Weatherhead 1984, Brodsky et al. 1988; but see D'Eon et al. 1994, Hoysak and Ankney 1996, McAuley et al. 1998). Our mtDNA data from Asia do not support that hypothesis but suggest instead that the opposite pattern is occurring in Asia.
Migration and dispersal of one group of organisms into the breeding range of another group are expected to increase gene flow and have a homogenizing effect on phenotypic and genetic diversity (Mayr 1942, 1963); that idea has been used to explain patterns of waterfowl phenotypic diversity (Anderson et al. 1992). Moreover, most Holarctic migratory ducks that pair seasonally do so during winter away from the breeding grounds (McKinney 1992, Oring and Sayler 1992). Seasonal monogamy and winterpairing chronology, consequently, are expected to homogenize genetic diversity in waterfowl. However, female waterfowl are strongly philopatric (Rohwer and Anderson 1988). Males follow females to natal-breeding sites, and females frequently return to the same nesting site year after year (Coulson 1984, Anderson 1985, Kehoe et al. 1989). Thus, female philopatry and nest-site fidelity are expected to counteract the effects of migration, dispersal, seasonal monogamy, and winter-pairing chronology.
Asymmetric hybridization between spot-bills and Mallards in East Asia is probably explained, in part, by Mallards outnumbering spot-bills by a factor of 6–10 during the breeding season in Primorye (Polivanova 1971); it might also be explained by differences in female and male dispersal. The recent northwest range expansion of spot-bills has resulted in more overlap of breeding spot-bills and Mallards. If male spot-bills show less site fidelity and migrate farther north than female spot-bills into the leading edge of the expanding spot-bill breeding range, which increasingly overlaps the Mallard's range (most Mallards nest farther north than most spotbills), more male spot-bills might be expected to mate with female Mallards than vice versa. In contrast, more female spot-bills would be expected to nest closer to the historical spot-bill breeding range farther south and mate with spot-bills. Consequently, offspring of male spot-bills x female Mallards that migrate south and pair with spot-bills in the following breeding season might then be expected to asymmetrically move Mallard mtDNA into the spot-bill population. Waterfowl habitat destruction in the southern Russian Far East (e.g. Khanka Lake) may also lead to additional opportunities for interspecific hybridization by altering densities of spot-bills and Mallards breeding in the same degraded habitat.
In contrast to mtDNA, asymmetrical gene flow at the ODC-6 locus (as measured by the two-island migration model) and equal allele frequencies in spot-bills and Mallards that are biased towards the type 1 alleles probably reflect Ne differences between the two species. Because nuclear gene flow is mediated by females and males, ODC-6 is not expected to reflect dispersal differences between sexes. Both species also show excess heterozygotes, which is expected if hybridization is occurring, and the departure from Hardy-Weinberg equilibrium proportions is significant for spot-bills. We hypothesize that excess heterozygosity for spot-bills (and Mallards to a lesser extent) is being maintained by immigration of spot-bills and Mallards from regions of allopatry well to the south and north of Primorye. We predict that allopatric populations of spot-bills and Mallards in those regions possess haplotype and genotypic frequencies historically diagnostic of each species. Preliminary mtDNA data for 39 Mallards from other parts of East Asia and the Aleutian Islands are consistent with that hypothesis (I. V. Kulikova et al. unpubl. data), but spot-bills from Southeast Asia and nuclear loci are yet to be genotyped.
Conclusion
Our study is the first to identify two divergent mtDNA haplotype and nuclear DNA allele lineages in Asian mallards and indicates that spot-bills are more closely related to Mottled, American Black, and Mexican ducks than they are to Mallards. Our data also suggest that spot-bills and Mallards have hybridized extensively in the Russian Far East, though alternative hypotheses—such as ancestral polymorphism and incomplete lineage sorting—clearly warrant further testing. Nonetheless, excess heterozygosity and apparent bias in group A and B mtDNA haplotype ratios, effective population sizes, and migration rates for mtDNA and ODC-6 are consistent with the hypothesis that more male spot-bills mated with female Mallards and more spot-bills received ODC-6 alleles from Mallards than vice versa.
The conclusion that spot-bills are close relatives of the New World monochromatic mallard species—Mottled, American Black, and Mexican ducks—is a new finding, but it is not surprising given the overall plumage similarities of those four species. However, it raises questions about the relationships of A. zonorhyncha to the Spot-billed Ducks (A. p. poecilorhyncha, A. p. haringtoni). Various authors have groupified A. zonorhyncha either as a subspecies of Spot-billed Duck (A. p. zonorhyncha; Johnsgard 1978, Sibley and Monroe 1990) or as a separate species (A. zonorhyncha; Phillips 1922-1926). Johnson and Sorenson (1999) sequenced one A. zonorhyncha and A. poecilorhyncha and found that both species possessed group A mtDNA haplotypes. Our results suggest that the A. zonorhyncha that Johnson and Sorenson (1999) sequenced acquired the group A mtDNA haplotype by hybridization with a Mallard, but what about their A. poecilorhyncha (which also was a captive aviary duck)? Is the Spot-billed Duck also a close relative of the New World mallard species? The systematic relationships of the mallard species and their mtDNA haplotype and nuclear DNA genotype frequencies in Asia, Europe, the Pacific, and the New World clearly warrant more investigation.
Acknowledgments
We thank V. A. Nechaev, Y. N. Glushenko, O. Burkovsky, and D. G. Pikunov for providing feather samples; and R. J. Harrigan, A. A. Nazarenko, and M. D. Sorenson for helpful suggestions. D. Haukos, S. Sonsthagen, and two anonymous reviewers provided helpful comments on the manuscript. The study was funded by the Institute of Biology and Soil Science, Far East Branch of the Russian Academy of Sciences, and the Institute of Arctic Biology and Alaska EPSCoR (National Science Foundation EPS-0092040) at the University of Alaska Fairbanks. Waterfowl were collected with shotguns and exported and imported in accordance with Russian and U.S. state and federal regulations and University of Alaska Fairbanks IACUC protocol 02-01.
Literature Cited