-
PDF
- Split View
-
Views
-
Cite
Cite
Scott A. Tarof, Laurene M. Ratcliffe, Habitat Characteristics and Nest Predation do not Explain Clustered Breeding in Least Flycatchers (Empidonax Minimus), The Auk, Volume 121, Issue 3, 1 July 2004, Pages 877–893, https://doi.org/10.1093/auk/121.3.877
- Share Icon Share
Abstract
Animals often exhibit territorial spatial structure in their breeding habitat. This clustering behavior is not well understood. We reviewed eight hypotheses for clustering and tested two ecological hypotheses for the formation of dense, territorial clusters in the Least Flycatcher (Empidonax minimus), a socially monogamous forest bird. The material resources hypothesis suggests that clustering is a response to habitat heterogeneity in vegetation, food, or both. The predation hypothesis proposes that clustering may reduce nest predation. Univariate and multivariate analyses of 170 vegetation plots from 1997 to 1998 indicated that forest-stand structure and tree species composition could not explain clustering in our population (predictions 1–3). Comparison of mean arthropod biomass inside with arthropod biomass outside two clusters sampled in 1999 using Malaise traps revealed that potential food resources were also unrelated to clustering (prediction 4). Nest predation rates were not correlated with territory position in clusters or with cluster size. In addition, predation rates were similar for clustered and solitary pairs (predictions 5–7). We conclude that habitat characteristics and nest predation do not explain clustered breeding in Least Flycatchers, though further tests of those hypotheses would be helpful. We develop the idea that the pursuit of extrapair copulations may promote clustered breeding. Future studies of territorial spatial structure in Least Flycatchers and other species should consider explanations based on mating behavior concomitant with ecological explanations for clustering.
RESUMEN
Los animales muchas veces exhiben estructura espacial territorial en sus ambientes de cría. Sin embargo, el comportamiento de agregación no se comprende claramente. En este estudio revisamos ocho hipótesis de agregación y evaluamos dos hipótesis ecológicas sobre la formación de agregados territoriales densos en Empidonax minimus, un ave de bosque socialmente monógama. La hipótesis de recursos materiales sugiere que la agregación es una respuesta a la heterogeneidad ambiental de la vegetación, del alimento o de ambos. La hipótesis de depredación propone que la agregación puede reducir la depredación de los nidos. Análisis univariados y multivariados de 170 parcelas de vegetación desde 1997 a 1998 indicaron que la estructura del bosque y la composición de especies de árboles no explicarían la agregación en nuestras poblaciones (predicciones 1–3). La comparación de la media ± EE de la biomasa de artrópodos dentro y fuera de dos agregados muestreados en 1999 usando trampas Malaise reveló que los recursos alimenticios potenciales tampoco se relacionaron con los patrones de agregación (predicción 4). Las tasas de depredación de nidos no se correlacionaron con la posición de los territorios en los agregados o con el tamaño de los agregados. Adicionalmente, las tasas de depredación fueron similares para parejas agregadas o solitarias (predicciones 5–7). Concluimos que las características del ambiente y la depredación de nidos no explican la agregación reproductiva en E. minimus, aunque análisis posteriores de estas hipótesis podrían ser útiles. Desarrollamos la idea de que la búsqueda de cópulas extra-pareja puede promover la agregación reproductiva. Estudios posteriores de la estructura espacial de los territorios en E. minimus y en otras especies deberían explicar la agregación considerando aspectos del comportamiento de apareamiento y explicaciones ecológicas.
factors that generate territorial spatial structure in animals constitute an important topic in ecology (May 1999). We use “spatial structure” to connote aggregation of individuals in coordinate space, a usage common in studies of statistical spatial distribution of organisms (hereafter “clustering”) (Legendre et al. 1985, Legendre and Fortin 1989, Bowman et al. 2000). One of the more pronounced forms of spatial structure in animals is clustering of allpurpose territories (i.e. all breeding activities occur in the same territory), where individuals settle adjacent to breeding conspecifics to produce clusters of densely aggregated territories in areas of seemingly homogeneous habitat. Consequently, available habitat appears unsaturated. Clustered breeding occurs in a wide variety of territorial animals, including fish (Itzkowitz 1978), insects (Muller 1998, Sumpter and Broomhead 2000), reptiles (Stamps 1988), primates (reviewed in Treves 2000), and especially birds (reviewed in Stamps 1988, 1994; Muller et al. 1997). Investigation of this type of clustering can elucidate possible factors that are important in habitat use (occupancy of an area), habitat selection (choice of an area based on preferred characteristics), or both. Birds offer a tractable natural system for testing hypotheses for clustering because territorial behavior is typically overt, clusters can be measured accurately, and habitat characteristics can be quantified with reasonable effort.
In birds, habitat availability, breeding density, horizontal and vertical vegetative strata, food resources, and foraging strategies contribute to habitat use during breeding (Hartley 1953; MacArthur and MacArthur 1961; MacArthur 1964; Fretwell and Lucas 1970; Holmes and Robinson 1981; Robinson and Holmes 1982, 1984; reviewed in Hildén 1965; Cody 1985; Morse 1989) and wintering (Johnson and Sherry 2001). For example, Franzreb (1978) found that birds preferred Douglas fir (Pseudotsuga menziesii), white fir (Abies concolor), and Engelmann spruce (Picea engelmanni) over other available tree species with distinctly different growth forms. Bay-breasted Warblers (Dendroica castanea) have been shown to settle opportunistically within patches of spruce budworms (Choristoneura fumiferana; Morse 1989) or forest tent caterpillars (Malacosoma disstria; Sealy 1979) that cover larger areas than the birds' territories. Indeed, it is generally accepted that birds have broad habitat preferences, but factors influencing settlement patterns at smaller scales (clustering within a particular habitat type) remain unclear for many species of birds.
Eight main hypotheses for clustering in birds (and other animals) have been proposed (Table 1). The material resources hypothesis (Kiester and Slatkin 1974) proposes that birds cluster in response to discontinuities in distribution of ecological resources (vegetation or food). In species where ecological resources influence settlement patterns, one might predict that clusters would form where nesting sites or food resources were locally abundant. That idea has been invoked to explain settlement patterns in Phylloscopus Warblers, Goldcrests (Regulus regulus; Tiainen et al. 1983, Morse 1989), Ruby-throated Hummingbirds (Archilochus colubris; Miller and Nero 1983), and Red-winged Blackbirds (Agelaius phoeniceus; Yasukawa and Searcy 1995), among others (see also MacArthur and MacArthur 1961, James 1971, James and Wamer 1982, Sherry and Holmes 1985). The predation hypothesis (Hamilton 1971, Pulliam 1973) suggests that clustering can reduce predation on adults or nests through proximate antipredator mechanisms. For example, dilution reduces predation on individual Common Redshanks (Tringa totanus) in wintering flocks (Cresswell 1994). Red-winged Blackbirds reduce nest predation through group vigilance and cooperative defense involving a unique alarm-call system that enhances communication among cluster members (Beletsky et al. 1986). Those two hypotheses are among the most common explanations for clustering; our aim was to examine them in the Least Flycatcher (Empidonax minimus).
Eight hypotheses for clustered breeding in territorial animals. Hypotheses are described in the context of clustered breeding in birds that defend all-purpose territories but likely apply to other animals as well. The information center hypothesis is unlikely to explain clustered breeding in such bird species because they typically feed in the same area in which the nest is located; that hypothesis is likely to be more applicable to colonial birds that forage outside the nesting colony.
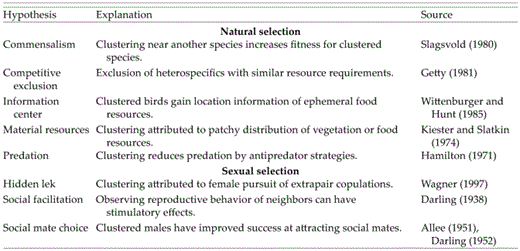
Eight hypotheses for clustered breeding in territorial animals. Hypotheses are described in the context of clustered breeding in birds that defend all-purpose territories but likely apply to other animals as well. The information center hypothesis is unlikely to explain clustered breeding in such bird species because they typically feed in the same area in which the nest is located; that hypothesis is likely to be more applicable to colonial birds that forage outside the nesting colony.
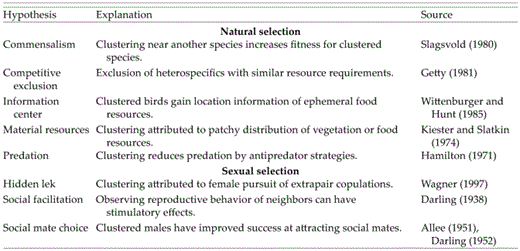
Least Flycatchers are migratory, socially monogamous birds that breed in mixed deciduous forests throughout North America. Least Flycatchers are known for their proclivity to cluster. In our population, most males defend small, all-purpose territories (0.1 ± 0.01 ha [SE], n = 101) with contiguous boundaries in dense clusters, while large areas of seemingly suitable forest remain unoccupied. Clustering is ubiquitous throughout their breeding range (MacQueen 1950, Davis 1959, Sherry and Holmes 1985, Perry and Andersen 2003), though some males settle solitarily (DellaSala and Rabe 1987, Tarof 2001). Ecological explanations for clustering in the species have been examined previously but our treatment of the subject is different in several ways. The spatial scale of vegetation sampling used by Sherry and Holmes (1985) was coarser than that used here. Perry and Andersen's (2003) study was based on an unbanded population with estimated territory boundaries. Moreover, vegetation analyses in Perry and Andersen (2003) were based on univariate comparisons only. We compared vegetation characteristics at three scales: (1) inside versus outside clusters within a forest, (2) occupied versus unoccupied habitat in different forests, and (3) clustered versus solitary territories. No other published study has tested for vegetation effects on Least Flycatcher clustering using this multilevel approach.
Multiple factors could act in concert to promote clustering in Least Flycatchers. For example, we have found mixed support for the idea that clustering may be related to female pursuit of extrapair copulations (EPCs) (Tarof 2001; see below). We tested seven predictions of the material resources and predation hypotheses. If vegetation characteristics (tree density, tree species composition, or both) influence clustering, we predicted (1) habitat inside clusters to be characterized by either higher tree density or species composition (or both) of sugar maple (Acer saccharum) trees than habitat outside clusters. This prediction is based on data that suggests that Least Flycatchers tend to be more abundant in areas where sugar maple trees are common, possibly for nesting sites (Darveau et al. 1992, Tarof 2001). We predicted the same differences in vegetation (2) between occupied and unoccupied forests and (3) between areas occupied by clustered and solitary males. If food availability affects settlement, then we expected (4) arthropod biomass to be higher inside than outside clusters. If clustered breeding reduces nest predation, we predicted predation rates (5) to be lower in central than in peripheral territories within clusters, (6) to correlate negatively with cluster size, and (7) to be higher for solitary pairs than for pairs in clusters.
Methods
Study area
We studied Least Flycatchers at Queen's University Biological Station (QUBS) (44°34′N, 76°19′W) near Kingston, Ontario, Canada from 1 May to 31 July 1997-2000. Queen's University Biological Station is a 2,200-ha research area comprising mixed deciduous forests mottled with lakes, wetlands, and fields. The three dominant tree species of the forest canopy are sugar maple, ironwood (Ostrya virginiana), and white ash (Fraxinus americana). The relatively open understory consists primarily of saplings of the three dominant canopy-tree species, as well as various shrubs and grasses. Forests in the area range in size from small patches (∼1 ha) to large contiguous forests. Within those forests, systematic changes in vegetation characteristics are not overt, but canopy openings do occur. Changes in either slope or aspect (or both) are generally minimal.
We monitored 21 clusters as part of a larger study of the spatial dynamics of clustering and social and genetic mate choice (Tarof 2001). Data presented here are from 8 of 21 clusters where habitat was sampled. Site descriptions appear in Table 2. Lower Bedford, Upper Bedford, and Skycroft were part of the same forest; the remaining five sites were separate (Table 2). Five paired solitary males' territories were also sampled (Table 2). Clusters ranged in size from 2 to 30 territories per cluster (7.4 ± 1.4) and occurred at densities of up to 11 territories ha−1. We defined clusters as aggregations of two or more territorial males with contiguous boundaries in the same forest and separated from other conspecifics in the same (or different) forest. Distance between clusters averaged 1,244.6 ± 200.8 m (range: 345.8–2,402 m). We defined solitary males as birds with no conspecific neighbors for at least 200 m. Average distance between a territorial solitary male and the nearest other male was 693.1 ± 108.2 m (range: 312.4–2,139 m).
Eight Least Flycatcher sites at Queen's University Biological Station (QUBS) and nearby areas where habitat was sampled from 1997 to 1999. Estimated forest sizes were as follows: Lower Bedford, Upper Bedford, and Skycroft (109.71 ha); Lower Lindsay Lake (30.09 ha); QUBS (28.11 ha); Roadside (1.20 ha); Upper Lindsay Lake (93.62 ha). Years in which clusters or solitary males were sampled reflect site occupancy.
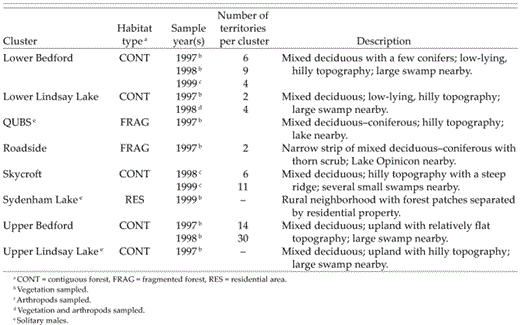
Eight Least Flycatcher sites at Queen's University Biological Station (QUBS) and nearby areas where habitat was sampled from 1997 to 1999. Estimated forest sizes were as follows: Lower Bedford, Upper Bedford, and Skycroft (109.71 ha); Lower Lindsay Lake (30.09 ha); QUBS (28.11 ha); Roadside (1.20 ha); Upper Lindsay Lake (93.62 ha). Years in which clusters or solitary males were sampled reflect site occupancy.
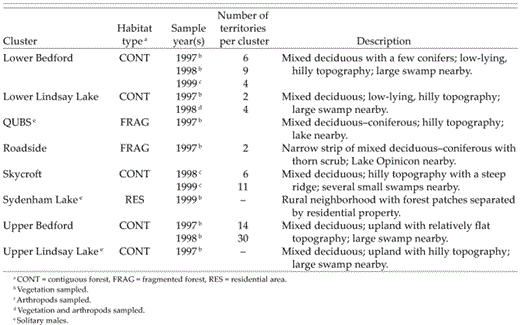
Study species
We captured adults by using playback and decoy between two mist nets or by erecting multiple nets at territory boundaries. We marked 98 adults with a Canadian Wildlife Service numbered band and a color band. We aged adults (second-year or after-second year) using rectrix shape (Pyle 1997) and measured body mass (to nearest 0.1 g), tarsus length, and flattened wing chord length (to nearest 0.1 mm). Females build nests, incubate eggs, and brood nestlings; both sexes feed offspring. In our population, males and females appear to choose nest trees together. Nests are typically found in sugar maple trees, but we have not analyzed data on nest-site characteristics to test whether sugar maples are chosen out of proportion to other available nest tree species (S. A. Tarof unpubl. data). Adults feed on small arthropods by “flycatching” (Briskie 1994) or “hovering” and taking prey from leaves in the forest canopy (Sherry 1979, Holmes and Robinson 1981). Nest predators include Blue Jays (Cyanocitta cristata), chipmunks (Tamias striatus), and black rat snakes (Elaphe obsoleta) (Briskie 1994). Males and females pursue EPCs (Tarof and Ratcliffe 2000, Tarof 2001).
Territory mapping
In June, we obtained Universal Transverse Mercator (UTM) coordinates (datum NAD 83 Canada, zone 18 North) for 101 territory boundaries in 10 of 21 clusters using a Trimble single-antenna Global Positioning System (GPS) unit attached to a TSC1 data logger with real-time correction (Trimble, Sunnyvale, California). The system allowed mapping to within 1 m accuracy during the peak period of breeding activity. Clusters were mapped identically. We used AUTOCAD MAP 2000 version 4.0 (Autodesk, San Rafael, California) and ARCVIEW version 3.2a (Environmental System Research Institute, Redlands, California) for Windows 95/98 to generate maps. We smoothed territory boundaries using “heads-up digitizing” without changing position or orientation. We mapped territories (not home ranges) of five solitary males.
Predictions 1–3: Clustering and vegetation
To examine the relationship between clustering and vegetation characteristics, we compared vegetation inside (n = 85 plots) and outside (n = 85 plots) five clusters in 1997 and three clusters in 1998 (n = 170 from eight clusters total) using 5-m radius (78.5 m2) plots. Sampling effort encompassed habitat used (inside cluster) and unused (outside cluster but in the same forest). In 1997, plots were located at 25-m intervals centered along linear transects. We selected transect direction so as to sample the greatest amount of habitat inside a cluster, while also sampling the unused habitat. We chose at random the starting position of each transect at the edge of clusters. A study on nest-site characteristics was initiated in 1998 (S. A. Tarof unpubl. data); we used control plots in territories from that data set as inside cluster plots. Plot locations (direction and distance from each nest plot) were determined at random, as were locations of plots outside clusters. For the two smallest clusters (Lower Lindsay Lake and Roadside; Table 2), vegetation was sampled 50 m beyond the outermost territory boundaries. Vegetation was sampled an average of 337.5 ± 86.5 m outside the other six clusters (range: 100–625 m).
Pseudoreplication in vegetation sampling was minimal. Only 3 of 98 (3%) banded adults in clusters returned during the four years of the study. One male returned to the same territory in a cluster in 1999 and 2000; two males returned to different clusters in 1999 than the clusters in which they were caught the previous year. Therefore, our population was made up almost exclusively of new birds each year. None of the three returning birds were in areas where habitat was sampled in more than one year. In addition, we flagged plot locations to avoid resampling the same point.

We determined percentage of canopy cover by averaging four readings (one per cardinal direction), using a spherical Model-A densiometer and multiplying number of canopy openings in the densiometer by 1.04 (Lemmon 1956). Difference between 100 and that value was the percentage of coverage (Lemmon 1956). We estimated percentage of ground cover in each plot by categorizing the proportion of cover by leaves and nonwoody shrubs on a scale of one (25% coverage) to four (100% coverage).
In addition to comparing vegetation inside with vegetation outside clusters in a forest, we compared stand structure of occupied and unoccupied sites (forests with Least Flycatchers versus separate forests without Least Flycatchers). We compared plots from inside three clusters (1997: Upper Lindsay Lake, Lower Bedford; 1998: Lower Lindsay Lake) with plots from forests unoccupied by Least Flycatchers since at least 1996 (Circle Trail and Seward R. Brown Field, n = 12 plots each). We restricted analyses to those three clusters to maintain an approximately equal number of plots. We included all structural variables in analyses, except percentage of canopy cover. Seward R. Brown Field had more trees than Circle Trail in the 6.41- to 11-cm DBH size class (z = 3.03, P = 0.003, Bonferroni critical α = 0.004, n = 12), but lower ratio of area:number of trees (t = 3.46, P = 0.002); therefore, we compared unoccupied sites with each cluster separately. We chose plots from unoccupied sites at random for comparisons with plots inside clusters.
In our third scale of analysis, we compared stand structure in five solitary territories (n = 17 pooled plots) with that inside two clusters (Upper Bedford 1997 and 1998). We chose those two clusters because they were the most representative areas of occupied Least Flycatcher habitat. We selected plots from inside clusters as described above. We based comparisons on all structural variables except percentage of canopy cover and ground cover (leaves). We were limited to five territories because we were unable to map territories for other solitary males accurately.
Prediction 4: Clustering and arthropods
We used Malaise traps (Townes 1972) to compare arthropod dry biomass (g) inside with biomass outside clusters in 1999. Arthropod biomass is often used to estimate abundance (Burke and Nol 1998, Holloway and Barclay 2000). We chose Malaise traps (3-m-high, pyramidal construction with ethyl acetate as killing agent; Bioquip Corporation, Gardena, California) because of their efficacy in sampling aerial insects in studies of insectivorous birds (Johnson and Sherry 2001).
Because only eight traps were available, we could sample only two clusters (Skycroft, 10 territories; Lower Bedford, 2 territories; 2 traps inside and outside each cluster). Outside cluster traps were ∼100 m from the outermost territory boundary. Sampling started 3 May 1999 and finished 30 June 1999, encompassing most of the Least Flycatcher breeding season (n = 467 samples). We excluded one sample collected on 6 July because of technical problems. We operated traps 24 h in all-weather conditions and emptied them at ∼1900 hours daily Eastern Standard Time. We removed arthropods stuck to the inner wall of collection heads using a fine brush. We stored samples at room temperature in 70% ethanol. We chose trap locations at random. Intertrap distances were similar at Skycroft (214.2 ± 53.4 m) and Lower Bedford (225.6 ± 139.3 m) (P > 0.05).
We decanted samples onto Whatman no. 1 9-cm filter paper and vacuum-filtered for 1 min to remove excess ethanol. We identified arthropods by order, dried them at 50°C for 24 h, and determined dry mass (to nearest 0.0001 g) using a Metler AE 160 electronic balance. We categorized samples as inside or outside a cluster, and paired by cluster for analysis.
Predictions 5–7: Clustering and predation
To examine the relationship between nest predation for first nest attempts and position in clusters and how predation varied with cluster size, we monitored the success of 112 nests. We defined successful nests as those that fledged at least one offspring. We found nests during nest building or egg laying by tracking females from mid-May to mid-June. We checked nests within 12 m of the ground (about 60% of first nests and 35% of renests) every 2–3 days using an extendible mirror pole. Because eggs were collected in five clusters (manipulated clusters) in 1998–2000 as part of a study on extrapair paternity (Tarof 2001), we performed correlations between predation rate and cluster size in two ways. First, we calculated predation rates using predation events that occurred up to day 5 of incubation (prior to egg collection) to include nests from unmanipulated and manipulated clusters. Next, we repeated that analysis using nests from unmanipulated clusters only. We determined overall predation rates using as many nests as possible from all clusters. In comparison of predation rates for nests in clusters with those of solitary nests, we were limited to five solitary nests because pairing success of solitary males was extremely low (Tarof 2001). We found solitary nests and nests in clusters during the same period.
We excluded nest failures caused by storm damage (one in 1998). We included nests destroyed by a predator during building (one in 1998, two in 1999, two in 2000), and partial predation events (three in 1998, one in 1999). We considered nests of polygynous males independent in analysis of how predation rate varied with cluster size because our interest was in how number of neighbors affected predation on individual nests. We did not calculate the Mayfield index (Mayfield 1961, 1975), because nest height prevented us from being able to monitor all nests and some nests in some clusters were manipulated by egg collection. Our goal was not to estimate nesting success per se, but to relate predation to territory settlement.
Data analysis
We used square-root transformations on number of live trees and number of saplings to achieve normality. Log-transformations normalized total basal area, area:number of trees ratio, and Simpson's diversity index (Sokal and Rohlf 1995). We used log (1 + x) transformations in principal components analyses (PCA, see below). To help interpret results from arthropod analyses, we estimated power of performed tests and least significant number (LSN) using observed effect sizes and standard errors (SEs). Least significant number was the number of observations needed to achieve a significant result, assuming the SE and effect size of the sample remained the same. We interpreted large LSN values as implying low statistical power to detect a meaningful relationship because of small sample size. Tests were 2-tailed. We applied Bonferroni corrections to minimize type I error (Rice 1989).
In addition to univariate comparisons for eight clusters, we used PCAs on correlation matrices to evaluate stand structure and tree species composition. We used PCAs in this analysis for two reasons. Our objective was to reduce a large number of variables into representative orthogonal linear components that maximized variation in the original variables and that could be presented in two-dimensional graphs (James and McCullough 1990, Legendre and Legendre 1998). In addition, we wanted to compare vegetation characteristics inside clusters relative to available habitat adjacent to clusters, without necessarily predicting, per se, where clusters might form—using linear discriminate functions, for example (James and McCullough 1990). Thus, we avoided problems of comparing mean and variance of a well-defined group (inside a cluster) with those of a poorly defined group (outside a cluster) (James and McCullough 1990, Ramsay et al. 1999). We performed PCAs on plots outside clusters to characterize the available habitat, while generating eigenvectors for all plots. We then plotted scores from the first two principal components (PC1 and PC2) with 95% density ellipses. Next, we compared PC scores of those two groups using post-hoc t-tests (means) and Bartlett's test (variances). We retained principal components with eigenvalues >1.0 (Kaiser 1960) but plotted PC1 and PC2 axes only because those two components together explained up to 77% (forest-stand structure) and 66% (tree species composition) of the total variance. That approach is similar to the recommended “percentage of variance” criterion of 75% (Bryant and Yarnold 1995).
To simplify PCAs, tree species were combined into 12 groups: sugar maple, “other maples” (black maple [A. nigrum], red maple [A. rubrum]), ironwood, basswood (Tilia americana), American elm (Ulmus americana), snags, birches (paper birch [Betula papyrifera], yellow birch [B. alleghaniensis]), hickory-ash (bitternut hickory [Carya cordiformis], shagbark hickory [C. ovata]), oaks (red oak [Quercus rubra], white oak [Q. alba]), beeches (American beech [Fagus grandifolia], blue beech [Carpinus caroliniana]), conifers (Eastern white cedar [Thuja occidentalis], Eastern hemlock [Tsuga canadensis], red pine [Pinus resinosa], white pine [P. strobus]), and “others” (prickly ash [Zanthoxylum americanum], apple [Malus pumila], large-tooth aspen [Populus grandidentata], black cherry [Prunus serotina], staghorn sumac [Rhus typhina], tamarack [Larix laricina], walnut [Juglans nigra]). We conducted PCAs on stand structure and tree species composition separately. The stand structure PCA related quantitatively to physical measures of the forest; species composition PCA related qualitatively to the suitability of nesting and foraging substrates. We excluded three small clusters (1997: Lower Lindsay Lake and Roadside; 1998: Lower Lindsay Lake) from those analyses because of insufficient numbers of plots sampled (n = 5).
Results
Vegetation
Our study area was predominantly deciduous (95% of total basal area; 5% coniferous). The three most common tree species were sugar maple (37%), ironwood (13%), and white ash (9%) (n = 27 species). Remaining deciduous tree species' abundances ranged from 0.002% to 8%.
In univariate comparisons (14 variables in 1997, 15 in 1998), one of the eight clusters showed differences in one stand structure variable. There were fewer small trees (3.3 ± 0.5) inside the 1998 Upper Bedford cluster relative to the adjacent habitat (6.04 ± 0.7) (z = 3.04, P = 0.002). No other parameters differed inside versus outside clusters (all P > 0.05, Bonferroni critical value α = 0.004). Similarly, there were no differences in stand structure between occupied and unoccupied Least Flycatcher sites (all P > 0.05, Bonferroni critical value α = 0.004). When plots from clusters were compared with those from solitary territories, only percentage of shrub cover was greater inside the 1998 Upper Bedford cluster (71.3 ± 6.5%) compared with solitary territories (39 ± 5.1%) (z = −3.3, P = 0.001, n = 17); other comparisons were nonsignificant (all P > 0.05).
Principal components analysis effectively combined the 14 or 15 stand structure variables into three orthogonal components that together explained 68–83% of the total variance in the original variables among the five clusters. The first component explained 29–49% of the total variance and loaded strongly with size and number of live trees for each cluster, except the 1997 Upper Lindsay Lake site (see below). The second component explained another 20–28% of the total variance in stand structure, loading with tree basal area, area:number of trees ratio, number of saplings, and number of snags. The third component explained an additional 12–20% of the total variance, loading strongly with tree species diversity. Thus, the three components corresponded to (1) tree size and density, (2) stand age and understory development, and (3) tree species diversity. In contrast, at the Upper Lindsay Lake cluster sampled in 1997, PC1 corresponded to tree density and stand age. Lindsay Lake was selectively logged more recently than the other four clusters, which may explain that difference in PC1 loadings (an older forest with fewer large trees). Plotting the first two PC axes revealed no differences in the mean or variance of stand structure for four of the clusters (all P > 0.05; Figs. 1A, B, C, E). The 1998 Upper Bedford site had fewer small trees inside the cluster (PC1: t = 0.04, P = 0.97, Bartlett's F = 3.3, P = 0.07; PC2: t = −3.8, P = 0.0004, Bartlett's F = 6.5, P = 0.01; Fig. 1D), as found with univariate comparisons. Lack of small trees may be explained by an ice storm that caused severe damage to the area in 1998, but that did not appear to deter settlement in the subsequent breeding season (Jones et al. 2001).
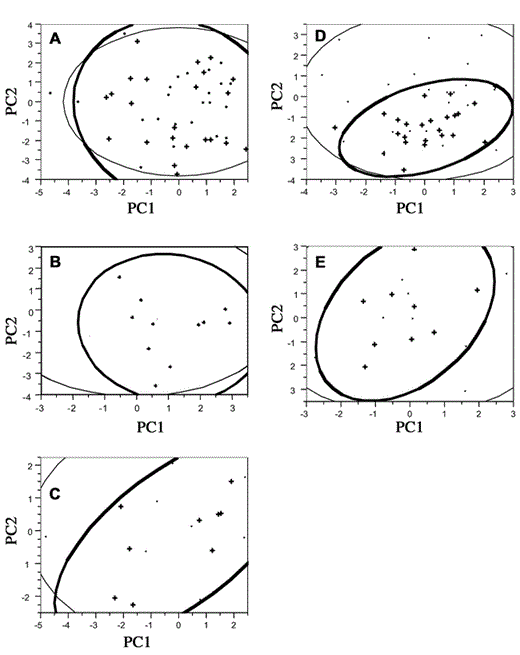
Principal components (PC) analysis of stand structure for five Least Flycatcher clusters: (A) Upper Bedford 1997, (B) Lower Bedford 1997, (C) Upper Lindsay Lake 1997, (D) Upper Bedford 1998, and (E) Lower Bedford 1998. Strong positive loadings correspond to habitat characteristics, such as larger tree size or density (see text). Density ellipses show 95% confidence space. Habitat inside clusters is represented by cross symbols and outlined with a thick line; adjacent habitat is shown with dots outlined with a thin line. Numbers of plots per cluster are 46, 22, 18, 50, and 18, respectively.
The PCA for tree species composition reduced the 12 species groups (see above) into 3 orthogonal components that collectively explained 61–84% of total variance in the original species of trees among the 5 clusters. The first component explained 23–36% of the total variance in tree species composition and was related mostly to sugar maple, ironwood, and birch trees. The second component explained 19–28% of the total variance, loading with American elm, beeches, and oaks. The third component explained another 17–23% of the total variance, loading with basswood, hickory-ash, and conifers. There were no overall differences in tree species composition inside compared with outside clusters (all P > 0.05; Fig. 2). In 1998, the Upper Bedford cluster appeared to contain an excess of ironwood and basswood trees, but that was attributed to an outlier (PC1: t = 0.28, P = 0.78, Bartlett's F = 1.8, P = 0.18; PC2: t = 2.6, P = 0.01, Bartlett's F = 7.4, P = 0.01; Fig. 2D). Together, these results suggest that vegetative features do not appear to affect clustering in Least Flycatchers (predictions 1–3).
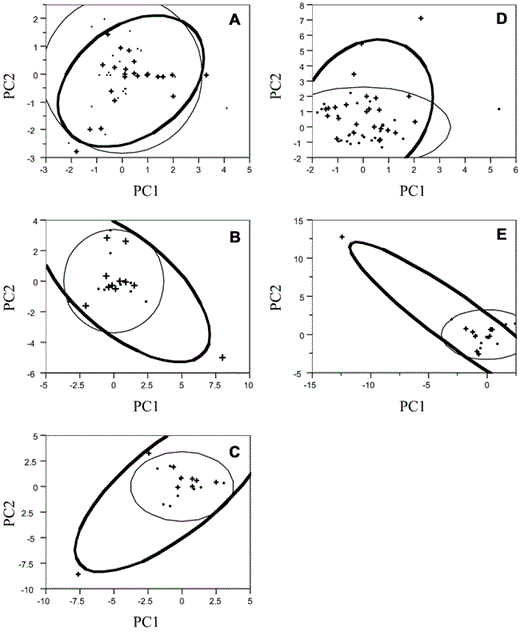
Principal components (PC) analysis of tree species composition of the five Least Flycatcher clusters in Figure 1. Strong positive loadings correspond to particular species composition characteristics, such as abundance of sugar maples, ironwood, or birches (see text). Density ellipses show 95% confidence space.
Arthropods
Contrary to prediction 4, arthropod biomass did not differ inside compared with outside clusters (all P > 0.05; Fig. 3); but the power of the analysis was low (Bedford: power = 0.08, LSN = 2,192; Skycroft: power = 0.28, LSN = 363). When we compared arthropod biomass inside with arthropod biomass outside clusters in 1999 only during the period when most males were settling in clusters (approximately 10–31 May), we found no difference (P = 0.45). Abundance peaked mid-May and early June inside and outside clusters, which was attributed mostly to an influx of dipterans (Fig. 4).
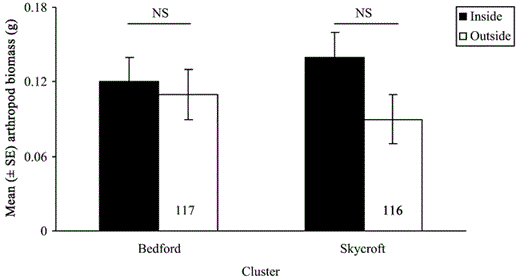
Arthropod biomass (mean ± SE) for Bedford (z = −0.6, P = 0.54, n = 234) and Skycroft (z = −1.1, P = 0.29, n = 233) clusters sampled from 3 May to 30 June 1999 (112,045 arthropods).
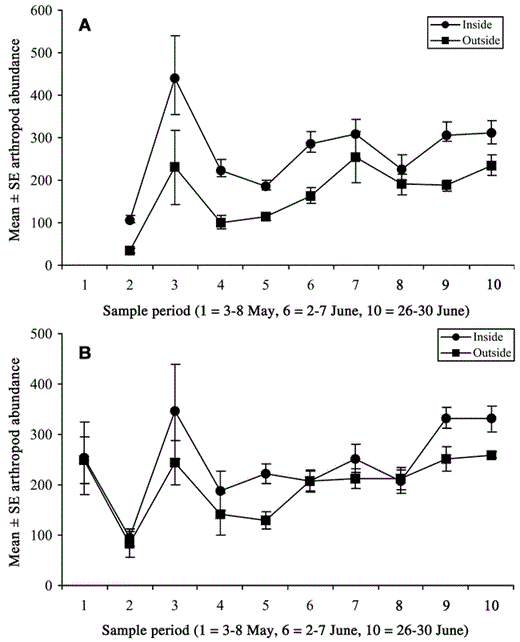
Arthropod phenology inside (circles) and outside (squares) (A) Bedford (n = 234 samples) and (B) Skycroft (n = 233 samples) Least Flycatcher clusters. Sampling period was divided into 6-day sessions from 3 May to 30 June. Collections began one week later at Bedford. Four Malaise traps were used per site.
Predation
There were no year effects in nest-predation rates (P > 0.05). Predation rates for first nests (49.1 ± 11.1%) and renests (48.2 ± 12.6%) were similar (F = 0.004, P = 0.95, nfirst = 112, nsecond = 24); but rates were higher during the egg stage compared to the nestling stage (F = 11.2, P = 0.007) (no interaction, F = 0.5, P = 0.51). Nest predation in clusters was unrelated to territory position (based on number of adjacent territories) in a logistic regression (χ2 = 2.3, P = 0.13, n = 58 nests from 10 clusters). There was no relationship between predation and number of territories per cluster, regardless of whether predation rates were based on the first five days of incubation for unmanipulated and manipulated clusters (rs = 0.05, P = 0.86, n = 13 clusters) or on unmanipulated clusters only (rs = −0.03, P = 0.96, n = 7 clusters). Predation rates for nests in clusters (55 ± 11.2%) compared with solitary nests (60 ± 18.1%) were similar (z = 0.15, ncluster = 13, nsolitary = 5, P = 0.88). These results indicated that predation rates did not vary with territory position within clusters or with cluster size, nor were predation rates higher for solitary pairs compared with pairs in clusters (predictions 5–7). Anecdotal behavioral observations of Least Flycatcher responses to Blue Jays near focal nests suggested some level of cooperative defense involving up to six neighboring adults (n = 20 cases).
Discussion
Vegetation and clustering
We did not detect vegetative heterogeneity that could explain clustering based on the first two scales of analysis (inside versus outside clusters, occupied versus unoccupied sites). Other studies have similarly found little or no support for the material resources hypothesis involving vegetation in Least Flycatchers (Sherry and Holmes 1985, Perry and Anderson 2003) and other species (Song Sparrow [Melospiza melodia], Nice 1937; Kirtland's Warbler [Dendroica kirtlandii], Morse 1989; Lazuli Bunting [Passerina amoena], Greene et al. 1996). Perry and Andersen (2003) compared 51 vegetative features inside with features outside Least Flycatcher clusters in Minnesota using univariate comparisons. The only difference detected was that percentage of cover by forbs and grasses was lower inside clusters than outside clusters. When we applied Bonferroni corrections to Perry and Andersen's (2003) results, that difference was nonsignificant. Sherry and Holmes (1985) sampled vegetation inside and outside clusters of Least Flycatchers breeding at Hubbard Brook, New Hampshire and found no differences. Our third scale of analysis (clustered versus solitary territories) also did not support the material resources hypothesis, but our ability to detect differences in that analysis was low because of small sample sizes. That cluster locations in our population were highly ephemeral (20 of 21 clusters were in different locations among years) further suggests that Least Flycatchers were not settling in response to vegetation (Tarof 2001). Although we might have detected some differences in vegetation had we sampled farther from clusters within forests (beyond 625 m), the apparent uniformity of the habitat throughout those forests makes that unlikely. Furthermore, our vegetation results were based on three distinct spatial scales, which is one of the strengths of our test of the material resources hypothesis.
Despite our findings, previous studies examining species abundance (not clustering per se) have shown Least Flycatchers to be influenced positively by stand structure, such as dense canopy, large or tall trees, and relatively open understory (Breckenridge 1956, DellaSala and Rabe 1987, Darveau et al. 1992). In their Hubbard Brook study, Holmes and Robinson (1981) concluded that variation in tree species did not explain patterns of Least Flycatcher abundance. Consequently, it appears that, at some spatial scales, Least Flycatchers exhibit some habitat preferences. However, those preferences cannot explain the consistent and tightly clustered breeding aggregations within contiguous, relatively homogeneous forests in our population.
Arthropods and clustering
The material resources hypothesis was not supported in terms of food resources. Results suggested that variation in arthropod biomass did not influence Least Flycatcher settlement. Perry and Andersen (2003) compared arthropod abundance, size, and dry weight inside and outside Least Flycatcher clusters using 40 sticky traps in each of eight clusters from mid-June to mid-July 1996 in Minnesota and also found no differences. To our knowledge, that is the only other study that has assessed arthropod abundance as a possible explanation for clustered breeding in this species.
Nonetheless, our arthropod results should be interpreted with caution. Sampling was limited to two clusters in a single year. Data from additional clusters in more than one year—and larger sample sizes for those clusters (based on power analyses)—would have improved the strength of our test of the material resources hypothesis. Moreover, the relationship between food availability and vegetative characteristics is complex (Holmes et al. 1979, Holmes and Robinson 1981, Holmes and Schultz 1988, Hutto 1990). Arthropod availability can vary with tree species, foliage structure (leaf position on branch, petiole length), and vertical strata; and birds can be sensitive to that variation. Least Flycatchers generally feed in the forest canopy, though not exclusively (Breckenridge 1956, Sherry 1979, Holmes and Robinson 1981). Although birds in our study sometimes foraged in the understory, canopy sampling may have targeted potential prey items more appropriately. We compared the three most common arthropod orders in our samples with results from other sampling techniques and with emetic data to address that possibility. Our Malaise traps captured primarily dipterans (86%), hymenopterans (4%), and coleopterans (2%). Perry and Andersen's (2003) dry weight data from sticky traps hung 5 m above the forest floor identified the most common orders as Diptera (42.6%), Coleoptera (19.9%), and Trichoptera (11.5%). Adult emetic data from Beal (1912) showed the most common orders (by counts) to be Hymenoptera (41.1%), Coleoptera (21.4%), and Diptera (11.3%) (reviewed by Briskie 1994). Sampling arthropods in the forest canopy would have improved our study.
Holmes and Schultz (1988) and Hutto (1990) recommended that studies measuring food availability also quantify foraging behavior, such as attack rates, movement patterns, and whether birds obtain prey from leaves, petioles, branches, or all three. Foraging behavior data would have enabled us to relate results on arthropod biomass to how birds perceived and used food resources. Those behavioral data would also have further improved our ability to gauge the efficacy of our sampling protocol. Finally, the scale of our arthropod sampling (100 m outside clusters) may have limited our ability to detect differences between used and unused habitat.
Predation effects on clustering
We found no support for the predation hypothesis. In contrast, Perry and Andersen (2003) found that central territories had lower nest predation rates than peripheral territories. Perry and Andersen (2003) also reported greater and faster responses by several nesting passerines (including Least Flycatchers) to a tethered hawk inside Least Flycatcher clusters than outside clusters. That differential response was attributable primarily to the high frequency of alarm calls and mobbing by Least Flycatchers. Perry and Andersen (2003) concluded that predation may be an important selective force promoting clustering.
Least Flycatchers are aggressive toward nest predators and Brown-headed Cowbirds (Molothrus ater) (Briskie 1994). Alarm calls may function as a predator “confusion” tactic (Curio 1978, Ficken 1989) that could be advantageous because more Least Flycatchers would be available to give alarm calls, possibly confusing predators to a greater extent. We propose two testable predictions of that idea. (1) Strength of alarm-call responses (and nesting success) should correlate positively with cluster size. (2) Nest survival of other passerines nesting in Least Flycatcher clusters should be higher compared with the nesting success of those same species outside clusters (see also Perry and Andersen 2003). Our ability to test the predation hypothesis was limited because of modest sample sizes. Given Perry and Andersen's (2003) findings and the coordinated mobbing behavior initiated by Least Flycatchers in our study, we recommend that the predation hypothesis be tested more rigorously in the future.
Alternative hypotheses for clustering
Several alternative hypotheses for clustered breeding in Least Flycatchers are possible. First, clustering may be in response to large-scale habitat features. In our study, 20 of 21 clusters (95%) formed adjacent to swamps (see also Perry and Andersen 2003). Swamps are likely important food sources for insectivorous birds, though arthropods may be distributed relatively evenly within a certain distance from a swamp edge. Interval arthropod sampling perpendicular to swamp edges will be needed to test that idea and evaluate the importance of clustering near swamps more completely. Seventeen of the 21 clusters (81%) in our population formed in areas of fairly homogeneous topography, which could improve cover from aerial predators during canopy flights or reduce foraging effort.
Second, clustering might be explained by the competitive exclusion hypothesis (Getty 1981; see Table 1). This hypothesis suggests that where two or more species share similar habitat requirements, the dominant species should aggregate to prevent heterospecifics from exploiting the habitat. Least Flycatchers and American Redstarts (Setophaga ruticilla) are forest birds that have converged on a feeding niche. They are similar in morphology (body size, bill size, and shape) and foraging behavior (“flycatching” or hovering for arthropods on foliage in the middle to upper canopy) (Sherry 1979, Holmes and Robinson 1981, Sherry and Holmes 1988), though Least Flycatchers are socially dominant to American Redstarts (reviewed by Briskie 1994). One might therefore predict Least Flycatchers to cluster their breeding territories during settlement, effectively pre-empting American Redstarts from using available habitat and food resources. Support for this hypothesis has been reported in several studies (Sherry 1979, Sherry and Holmes 1988, Martin et al. 1996). We did not test the competitive exclusion hypothesis, but American Redstarts rarely overlapped with Least Flycatcher clusters in our population, which is consistent with the idea of Least Flycatchers mediating habitat use by a competitively subordinate species with a similar ecological niche.
Third, Wagner (1997) has suggested that clustering may be related to extrapair mating strategies, an idea known as the “hidden lek hypothesis.” Leks are aggregations of males that females visit for promiscuous copulations. In many lekking species, females typically prefer to mate with males at the center of the lek (“hotshot males”) and avoid those displaying peripherally or solitarily, which produces a skew in male mating success (reviewed by Höglund and Alatalo 1995). The hidden lek hypothesis proposes that the mechanisms producing leks can similarly produce clusters of all-purpose territories in socially monogamous birds seeking extrapair copulations, where females engage in extrapair matings (Petrie and Kempenaers 1998, reviewed by Stutchbury and Neudorf 1998). If that is true, then three important lines of evidence need to be supported. (1) Males in clusters of all-purpose territories should be preferred as social mates; solitary males should be avoided. (2) Females should pursue EPCs, though males may also seek EPCs. (3) Patterns of extrapair paternity should reveal a skew in male mating success favoring central males.
From 1997 to 2001, we tested the hidden lek hypothesis using data on male settlement and arrival at the breeding grounds, male pairing success, male and female mating behavior, and patterns of extrapair paternity (Tarof 2001). We found that 87% (245 of 281) of clustered males paired whereas < 23% (10 of 44) of solitary males attracted a social partner (P < 0.0001). In clusters, central males arrived earlier (P = 0.0003), were heavier (P = 0.02) and in better body condition (P = 0.04), and had higher pairing success compared with peripheral males (P = 0.004). Observations of 51 pairs showed that EPCs were common in Least Flycatchers (see also Tarof and Ratcliffe 2000). Eleven fertile females solicited a total of 19 EPCs from neighboring males while on territory (copulation confirmed visually in two cases). Furthermore, 23% (6 of 26) of extraterritorial forays were by females. However, we could not show conclusively that those female forays were for obtaining EPCs or that female mating behavior promoted male clustering. Microsatellite data from 21 nests in clusters sampled in 1999 and 2000 indicated that extrapair paternity was more likely in peripheral nests, though we did not find the predicted positive correlation between territory position and genetic mating success favoring central males. In summary, our results offered mixed support for the idea that Least Flycatcher clusters in our population were analogous to hidden leks.
Finally, clustering might be explained by social mate choice. That hypothesis predicts that males should cluster to increase their success at attracting social partners (Allee 1951, Darling 1952). Results described above regarding female preference for clustered males over solitary males as social mates are consistent with the social mate-choice hypothesis. However, that hypothesis was unable to explain the relationship between male pairing success and centrality. Moreover, that hypothesis could not explain extrapair paternity being more common in peripheral nests.
Habitat selection
Our study does not imply that habitat cues are not important in habitat selection. Our objective was to examine ecological correlates of Least Flycatcher settlement, rather than to identify cues used when selecting breeding sites from among the available habitat (Cody 1985, Johnson and Sherry 2001). Habitat manipulations at various spatial scales will be necessary to examine habitat selection directly. For example, in a natural or experimental local eruption of arthropods, we would expect Least Flycatchers to respond predictably to such perturbations of food resource availability (Johnson and Sherry 2001).
Many studies, including the present one, have examined either material resources or predation (or both) as explanations for clustering in Least Flycatchers and have not found strong evidence for either hypothesis. Although our results do not allow us to rule out the possibility that clustering is related to habitat (at some spatial scales) or predation, the data available lead us to conclude that the hidden lek hypothesis is the most likely explanation for territorial spatial structure in our population. We consider the nonsignificant results reported here as making a positive contribution to current theory regarding what might be driving clustering in Least Flycatchers. We recommend that future studies examining ecological factors for clustering do so at various levels of spatial scale and simultaneously consider mating opportunities and competitive interactions with other species as potentially important social factors (Sherry and Holmes 1985, Tarof 2001). Potential fitness gains from clustering because of sociality may be additive with ecological factors. The hidden lek hypothesis has potentially far-reaching implications for identifying social factors that help constitute “ideal” breeding conditions for territorial animals.
Acknowledgments
J. Bos, N. Dinsley, H. Ducharme, T. Feroz, H. Holka, M. Kasumovic, and N. Vreeswyk assisted with fieldwork. Thanks to S. Zambon for processing arthropod samples and to E. Nol for Malaise traps. Research was conducted under Queen's University Animal Care approval and Environment Canada permits 10302-AR and CA-0033. Queen's University Biological Station provided logistical support. The work was supported by Natural Sciences and Engineering Research Council of Canada and Ontario Graduate Scholarship in Science and Technology, Frank M. Chapman Memorial Fund of the American Museum of Natural History, American Ornithologists' Union, John K. Cooper Foundation, and Society of Canadian Ornithologists grants to S.A.T., Queen's University, and NSERC grants to L.M.R. Contact S.A.T. for details of univariate comparisons of forest stand structure inside versus outside clusters.
Literature Cited