-
PDF
- Split View
-
Views
-
Cite
Cite
Yunheng Ji, Changkun Liu, Jacob B Landis, Min Deng, Jiahui Chen, Plastome phylogenomics of Cephalotaxus (Cephalotaxaceae) and allied genera, Annals of Botany, Volume 127, Issue 5, 9 April 2021, Pages 697–708, https://doi.org/10.1093/aob/mcaa201
- Share Icon Share
Abstract
Cephalotaxus is a paleo-endemic genus in East Asia that consists of about 7–9 conifer species. Despite its great economic and ecological importance, the relationships between Cephalotaxus and related genera, as well as the interspecific relationships within Cephalotaxus, have long been controversial, resulting in contrasting taxonomic proposals in delimitation of Cephalotaxaceae and Taxaceae. Based on plastome data, this study aims to reconstruct a robust phylogeny to infer the systematic placement and the evolutionary history of Cephalotaxus.
A total of 11 plastomes, representing all species currently recognized in Cephalotaxus and two Torreya species, were sequenced and assembled. Combining these with previously published plastomes, we reconstructed a phylogeny of Cephalotaxaceae and Taxaceae with nearly full taxonomic sampling. Under a phylogenetic framework and molecular dating, the diversification history of Cephalotaxus and allied genera was explored.
Phylogenetic analyses of 81 plastid protein-coding genes recovered robust relationships between Cephalotaxus and related genera, as well as providing a well-supported resolution of interspecific relationships within Cephalotaxus, Taxus, Torreya and Amentotaxus. Divergence time estimation indicated that most extant species of these genera are relatively young, although fossil and other molecular evidence consistently show that these genera are ancient plant lineages.
Our results justify the taxonomic proposal that recognizes Cephalotaxaceae as a monotypic family, and contribute to a clear-cut delineation between Cephalotaxaceae and Taxaceae. Given that extant species of Cephalotaxus are derived from recent divergence events associated with the establishment of monsoonal climates in East Asia and Pleistocene climatic fluctuations, they are not evolutionary relics.
INTRODUCTION
The economically important genus Cephalotaxus Siebold & Zuccarini ex Endlicher consists of about 7–9 evergreen coniferous species occurring in Burma, China, India, Japan, Korea, Laos, Malaysia, Thailand and Vietnam (Fu, 1984; Fu et al., 1999; Farjon, 2010; Lang et al., 2013; Yang, 2017; J. W. Zhang et al., 2019). According to fossil records, the earliest Cephalotaxus species was present in Europe by the middle Eocene (Manchester et al., 2009) and expanded its distribution range into East Asia (north-eastern China) by the late Eocene (He and Tao, 1997). During the Miocene and Pliocene, Cephalotaxus was widespread across the Northern Hemisphere in Eurasia and North America (Miki, 1958; Givulescu, 1973; Mai and Walther, 1978; Wilde 1989; He and Tao, 1997; Kvaček and Walther, 1998; Meller, 1998; Kvaček and Rember, 2000; Walther and Kvaček, 2007). Therefore, the extant members of the genus have a relatively restricted distribution possibly resulting from range contraction and southward migration driven by decreasing global temperatures of the Neogene and Pleistocene glaciation events (Axelrod, 1959; Qian and Ricklefs, 2000; Manchester et al., 2009). Because of its antiquity, Cephalotaxus is an excellent representative of relict plant lineages in East Asia (Manchester et al., 2009), and extant species within this genus are hypothesized to be evolutionary relicts (Fu, 1984; Wu et al., 2005).
Cephalotaxus is distinctive in having two-ovulate bracts in the seed cones (Fu et al., 1999); vegetative characters including general folia morphology and growth habit closely resemble those of Amentotaxus Pilger, Austrotaxus Compton, Pseudotaxus W. C. Cheng, Taxus Linn. and Torreya Arnott. Although the monophyly of Cephalotaxus has been robustly supported by both morphological (Ghimire and Heo, 2014) and molecular evidence (Cheng et al., 2000; Hao et al., 2008; Gao et al., 2015), its taxonomic affinities remain largely disputed. Historically, Cephalotaxus was placed into either Taxaceae (Eichler, 1889; Van Tieghem, 1891; Pilger, 1903; Takhtajan, 1953; Hart, 1987; Price, 1990; Stefanoviac et al, 1998; Christenhusz, 2011) or Cephalotaxaceae (Neger, 1907; Florin, 1948, 1954; Singh, 1961). Due to contradicting taxonomic proposals inferred from different evidence, it remains unresolved whether Cephalotaxaceae should be recognized as a separate family or merged back within Taxaceae. For instance, cladistic analysis based on morphological characters (Ghimire and Heo, 2014) as well as phylogenetic inference utilizing 18S ribosomal DNA sequences (Chaw et al., 1997) and plastid rbcL and matK regions (Quinn et al., 2002) suggest that Cephalotaxaceae should be merged into Taxaceae. However, phylogenetic analyses using a combination of plastid matK and nuclear ribosomal internal transcribed spacer (ITS) regions (Cheng et al., 2000), plastid matK, rbcL, trnL–trnF, psbA–trnH and nuclear ITS (Hao et al., 2008), nuclear LFY and NLY (Lu et al., 2014), and transcriptomic data (Majeed et al., 2018; Ran et al., 2018) support the sister relationship between Cephalotaxaceae and Taxaceae. Nevertheless, a clear-cut delineation between the two families remains unresolved due to the ambiguous position of Amentotaxus and Torreya. Although the analyses by Ran et al. (2018) and X. Zhang et al. (2019) showed that these two genera are closer to Taxaceae, Cheng et al. (2000), Hao et al. (2008), Lu et al. (2014) and Majeed et al. (2018) proposed to include them within Cephalotaxaceae. These conflicts necessitate that the relationships between Cephalotaxus and its relatives require further investigation.
Additionally, the traditional classification of Cephalotaxus is constructed primarily based on folia morphologies, including leaf size, shape of leaves and leaf base, density and arrangement of leaves, etc. (Fu, 1984; Fu et al., 1999; Farjon, 2010; Lang et al., 2013; Yang, 2017; J. W. Zhang et al., 2019). These characters are highly divergent, and are usually overlapping between species (Tripp, 1995), making morphology-based taxonomy particularly perplexing and challenging (J. W. Zhang et al., 2019). Since the establishment of the genus by Endlicher (1847), Cephalotaxus has been the subject of several taxonomic revisions (Fu et al., 1999; Farjon, 2010; Lang et al., 2013; J. W. Zhang et al., 2019). Yet, credible species delineation and unambiguous interspecific relationships within the genus remain unresolved. Given their great economic and ecological importance (Fu, 1984; Lang et al., 2013), resolving the aforementioned problems will help the utilization and conservation of extant Cephalotaxus species, and deepen our understanding of the evolutionary history of gymnosperms.
Phylogenetic reconstruction based on limited sequence variation often results in poor resolution and low support in relationships, particularly at lower taxonomic levels (Rokas and Carroll, 2005; Whitfield and Lockhart, 2007; Philippe et al., 2011). In contrast to Sanger sequencing, next-generation sequencing techniques, which are capable of generating orders of magnitude more data, have offered new approaches to resolving historical problems in plant phylogenetics (e.g. Parks et al., 2009; Barrett et al., 2014; Stull et al., 2015; Zhang et al., 2017; Carlsen et al., 2018; Dong et al., 2018; Li et al., 2019). Plastid genome (plastome) DNA sequences, which harbour a large number of evolutionarily informative variation suitable for phylogenetic analysis, have been widely employed to reconstruct robust evolutionary relationships in phylogenetically challenging plant taxa (e.g. Simpson et al., 2017; Uribe-Convers et al., 2017; Heckenhauer et al., 2019; Ji et al., 2019a).
Here, we sequenced and assembled whole plastomes of nine species of Cephalotaxus and two species of Torreya using a low coverage genome sequencing approach. Based on plastome phylogenomic analyses and fossil-calibrated molecular dating, we aim to (1) elucidate the relationships between Cephalotaxus and related genera; (2) investigate interspecific relationships within Cephalotaxus; and (3) infer the history of species diversification for Cephalotaxus.
MATERIALS AND METHODS
Plant materials, shotgun sequencing, plastome assembly and comparison
According to the most recent taxonomic revisions on Cephalotaxus (Lang et al., 2013; J. W. Zhang et al., 2019), our taxon sampling covered all seven species (Cephalotaxus alpina, C. fortunei, C. griffithii, C. hainanensis, C. harringtonii, C. nana and C. oliveri) recognized in the genus. We also sampled C. mannii and C. sinensis whose taxonomic status as a recognized species differ according to different authors (Fu et al., 1999; Farjon, 2010; Lang et al., 2013; J. W. Zhang et al., 2019), to investigate their relationships to their congeneric species. Voucher specimens (Table 1) were deposited at the herbarium of Kunming institute of Botany, Chinese Academy of Sciences (KUN).
Taxa newly sequenced in this study with source, voucher and GenBank accession numbers
Taxa . | Locality . | Voucher . | GenBank accession . |
---|---|---|---|
Cephalotaxus alpina | Zixishan Mountain, Chuxiong, Yunnan, China | Y. Ji and C. Tao 012 | MT555079 |
C. fortunei | Jinfoshan Mountain, Nanchuan, Chongqing, China | Y. Ji and C. Liu 084 | MT555080 |
C. griffithii | Dulongjiang, Gongshan, Yunnan, China | GLGS Exp. 38714 | MT555081 |
C. hainanensis | Jianfengling, Ledong, Hainan, China | T. Su s. n. | MT555082 |
C. harringtonii | Cultivated in the Botanical Garden of Kunming Institute of Botany | Y. Ji and X. Gong 002 | MT555083 |
C. mannii | Cultivated in the Botanical Garden of Kunming Institute of Botany | Y. Ji and X. Gong 003 | MT555084 |
C. nana | Fengxi, Zhuxi, Hubei, China | Y. Ji and C. Liu 095 | MT555085 |
C. oliveri | Cultivated in the Botanical Garden of Kunming Institute of Botany | Y. Ji and X. Gong 001 | MT555086 |
C. sinensis | Dengchigou, Baoxing, Sichuan, China | Y. Ji, W. Chen and T. Su 180 | MT555087 |
Torreya fargesii var. yunnanensis | Cultivated in the Botanical Garden of Kunming Institute of Botany | Y. Ji and X. Gong 005 | MT555088 |
T. jackii | Cultivated in the Botanical Garden of Kunming Institute of Botany | Y. Ji and X. Gong 004 | MT555089 |
Taxa . | Locality . | Voucher . | GenBank accession . |
---|---|---|---|
Cephalotaxus alpina | Zixishan Mountain, Chuxiong, Yunnan, China | Y. Ji and C. Tao 012 | MT555079 |
C. fortunei | Jinfoshan Mountain, Nanchuan, Chongqing, China | Y. Ji and C. Liu 084 | MT555080 |
C. griffithii | Dulongjiang, Gongshan, Yunnan, China | GLGS Exp. 38714 | MT555081 |
C. hainanensis | Jianfengling, Ledong, Hainan, China | T. Su s. n. | MT555082 |
C. harringtonii | Cultivated in the Botanical Garden of Kunming Institute of Botany | Y. Ji and X. Gong 002 | MT555083 |
C. mannii | Cultivated in the Botanical Garden of Kunming Institute of Botany | Y. Ji and X. Gong 003 | MT555084 |
C. nana | Fengxi, Zhuxi, Hubei, China | Y. Ji and C. Liu 095 | MT555085 |
C. oliveri | Cultivated in the Botanical Garden of Kunming Institute of Botany | Y. Ji and X. Gong 001 | MT555086 |
C. sinensis | Dengchigou, Baoxing, Sichuan, China | Y. Ji, W. Chen and T. Su 180 | MT555087 |
Torreya fargesii var. yunnanensis | Cultivated in the Botanical Garden of Kunming Institute of Botany | Y. Ji and X. Gong 005 | MT555088 |
T. jackii | Cultivated in the Botanical Garden of Kunming Institute of Botany | Y. Ji and X. Gong 004 | MT555089 |
Taxa newly sequenced in this study with source, voucher and GenBank accession numbers
Taxa . | Locality . | Voucher . | GenBank accession . |
---|---|---|---|
Cephalotaxus alpina | Zixishan Mountain, Chuxiong, Yunnan, China | Y. Ji and C. Tao 012 | MT555079 |
C. fortunei | Jinfoshan Mountain, Nanchuan, Chongqing, China | Y. Ji and C. Liu 084 | MT555080 |
C. griffithii | Dulongjiang, Gongshan, Yunnan, China | GLGS Exp. 38714 | MT555081 |
C. hainanensis | Jianfengling, Ledong, Hainan, China | T. Su s. n. | MT555082 |
C. harringtonii | Cultivated in the Botanical Garden of Kunming Institute of Botany | Y. Ji and X. Gong 002 | MT555083 |
C. mannii | Cultivated in the Botanical Garden of Kunming Institute of Botany | Y. Ji and X. Gong 003 | MT555084 |
C. nana | Fengxi, Zhuxi, Hubei, China | Y. Ji and C. Liu 095 | MT555085 |
C. oliveri | Cultivated in the Botanical Garden of Kunming Institute of Botany | Y. Ji and X. Gong 001 | MT555086 |
C. sinensis | Dengchigou, Baoxing, Sichuan, China | Y. Ji, W. Chen and T. Su 180 | MT555087 |
Torreya fargesii var. yunnanensis | Cultivated in the Botanical Garden of Kunming Institute of Botany | Y. Ji and X. Gong 005 | MT555088 |
T. jackii | Cultivated in the Botanical Garden of Kunming Institute of Botany | Y. Ji and X. Gong 004 | MT555089 |
Taxa . | Locality . | Voucher . | GenBank accession . |
---|---|---|---|
Cephalotaxus alpina | Zixishan Mountain, Chuxiong, Yunnan, China | Y. Ji and C. Tao 012 | MT555079 |
C. fortunei | Jinfoshan Mountain, Nanchuan, Chongqing, China | Y. Ji and C. Liu 084 | MT555080 |
C. griffithii | Dulongjiang, Gongshan, Yunnan, China | GLGS Exp. 38714 | MT555081 |
C. hainanensis | Jianfengling, Ledong, Hainan, China | T. Su s. n. | MT555082 |
C. harringtonii | Cultivated in the Botanical Garden of Kunming Institute of Botany | Y. Ji and X. Gong 002 | MT555083 |
C. mannii | Cultivated in the Botanical Garden of Kunming Institute of Botany | Y. Ji and X. Gong 003 | MT555084 |
C. nana | Fengxi, Zhuxi, Hubei, China | Y. Ji and C. Liu 095 | MT555085 |
C. oliveri | Cultivated in the Botanical Garden of Kunming Institute of Botany | Y. Ji and X. Gong 001 | MT555086 |
C. sinensis | Dengchigou, Baoxing, Sichuan, China | Y. Ji, W. Chen and T. Su 180 | MT555087 |
Torreya fargesii var. yunnanensis | Cultivated in the Botanical Garden of Kunming Institute of Botany | Y. Ji and X. Gong 005 | MT555088 |
T. jackii | Cultivated in the Botanical Garden of Kunming Institute of Botany | Y. Ji and X. Gong 004 | MT555089 |
Total genomic DNA for each species was isolated from approx. 20 mg of silica gel-dried leaf tissues using the CTAB (cetyltrimethylammonium bromide) method (Doyle and Doyle, 1987). Purified genomic DNA (approx. 5 µg) was used to construct shotgun libraries with a TruSeq DNA Sample Prep Kit (Illumina, Inc., USA) following the manufacturer’s instructions. Paired-end sequencing was conducted on the Illumina HiSeq 2500 platform. Shotgun reads were subjected to the NGS QC Toolkit (Patel and Jain, 2012) to remove adaptors and low-quality reads with default parameters. Based on the remaining high-quality Illumina reads, de novo plastome assembly was performed using NOVOPlasty v2.7.0 (Dierckxsens et al., 2017) with a k-mer of 31, and using the large subunit of the Rubisco gene (rbcL) of C. oliveri (AF456387) as the seed for iterative extension of contigs to recover the complete plastome of each species.
The newly generated plastomes were annotated with the Dual Organellar Genome Annotator database (Wyman et al., 2004); the annotation of protein-coding genes was confirmed with a BLAST search against the NCBI protein database. Genes putatively annotated as tRNA were further verified by tRNAscan-SE 1.21 (Schattner et al., 2005) with default parameters. To identify plastome arrangement events in Cephalotaxus, the newly generated plastomes were progressively aligned with those of four species representing closely related genera, Amentotaxus, Torreya, Pseudotaxus and Taxus, using the multiple genome alignment program Mauve 2.3.1 (Darling et al., 2010). Moreover, all Cephalotaxus plastomes were aligned pairwise using the program mVISTA (Mayor et al., 2000) under the LAGAN mode to investigate sequence divergence.
Phylogenetic analyses
In addition to the nine Cephalotaxus plastomes newly generated in this study, a previously sequenced plastome of C. wilsoniana, which was treated as a conspecific variety (Fu et al., 1999; Farjon, 2010) or a synonym of C. harringtonii (Lang et al., 2013; J. W. Zhang et al., 2019), was included in phylogenetic analyses. Moreover, six Cupressaceae plastomes, two Amentotaxus plastomes, eight Torreya plastomes, one Pseudotaxus plastome and 14 Taxus plastomes available in GenBank were incorporated into the dataset (Supplementary data Table S1). According to the backbone relationships of gymnosperms (Christenhusz et al., 2011; Ran et al., 2018), Agathis danunara and Podocarpus totara were selected as outgroups. Eighty-one plastid protein-coding genes commonly shared (Supplementary data Table S2) were aligned with MAFFT v7.4 (Katoh et al., 2013). The best-fitting partition scheme was selected using PartitionFinder v2.1.1 (Lanfear et al., 2017) with the ‘greedy’ search algorithm.
Both maximum likelihood (ML) and Bayesian inference (BI) approaches were used for phylogenetic inference. The ML analyses were conducted with RAxML-HPC BlackBox v8.1.24 (Stamatakis, 2006) with the most suitable model (GTR + G + I) for sequence substitution selected using Modeltest v3.7 (Posada and Crandall, 1998) with the Akaike information criterion (Posada and Buckley, 2004). Ten independent ML searches were performed with 1000 standard bootstrapping replicates. The BI analyses were performed using MRBAYES v 3.1.2 (Ronquist and Huelsenbeck, 2003). Markov chain Monte Carlo (MCMC) runs were initiated with a random tree for 1 million generations, with trees sampled every 100 generations. Trees from the first 250 000 generations were discarded as burn-in. The posterior probability (PP) values were computed based on the remaining trees.
Divergence time estimation
The program BEAST v. 2.4.7 (Bouckaert et al., 2014) was employed to estimate divergence time. We incorporated three fossils to calibrate the molecular clock. The crown age of the lineage including Cupressaceae, Cephalotaxaceae and Taxaceae was set to 197 million years ago (Mya) (Florin, 1958, 1963). The most recent common ancestor of Cephalotaxaceae and Taxaceae was set to a minimum age of 163.5 Mya (Florin, 1963). The stem age of Taxus was set to a minimum age of 100 Mya (Xu et al., 2015). We used the uncorrelated log-normal relaxed molecular clock approach, which allows uncertainty in the age of calibrations to be represented as prior distributions rather than as strict calibration/fixed points. The BEAST analyses were conducted under the sequence substitution model (GTR + G + I) selected by Modeltest with a Yule tree prior. Two independent replications of MCMC simulations were run, sampling every 1000 generations. The stationarity of the chains and convergence of MCMC simulations were monitored by Tracer v1.7.1 (Rambaut et al., 2018). After stationarity was obtained, a maximum credibility tree was constructed with TreeAnotator v1.10 (http://beast.bio.ed.ac.uk/TreeAnnotator), with the first 5000 trees discarded as burn-in.
RESULTS
Features of Cephalotaxus plastomes
Shallow-depth genome sequencing produced approx. 30.1–31.5 million paired-end clean reads per sample. Of those, 4.16 × 105–1.00 × 106 were mapped to the assembled plastomes, with the average sequencing coverage ranging from 464× to 1117× (Supplementary data Table S3). The de novo assemblies generated nine circular Cephalotaxus plastomes, with the sizes of the complete plastome, coding regions and non-coding regions varying from 134 550 to 136 545 bp, 82 928 to 83 985 bp and 51 622 to 52 707 bp, respectively (Supplementary data Table S4). All Cephalotaxus species possessed a similar GC content not only in the whole plastomes assembly (35.1–35.2 %), but also in coding (37.3–37.5 %) and non-coding (31.%–31.6 %) regions (Supplementary data Table S4). All Cephalotaxus plastomes identically encoded 113 unique genes, comprising four rRNA genes, 27 tRNA genes and 82 protein-coding genes (Supplementary data Table S5). In addition, due to the loss of the typical inverted repeat region, it is difficult to define the boundary of large single-copy and small single-copy regions in Cephalotaxus plastomes (Fig. 1).
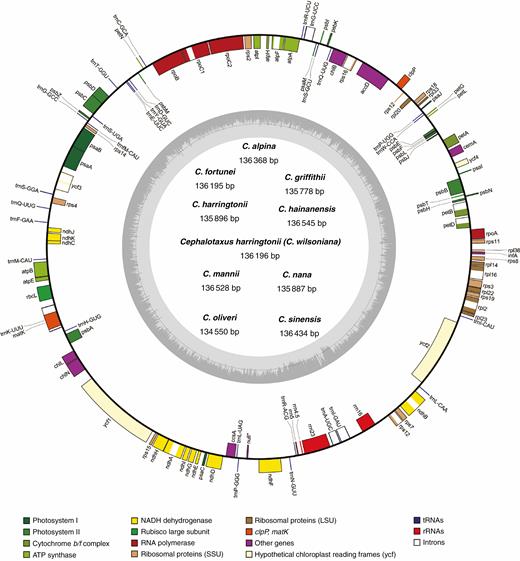
Map of Cephalotaxus plastomes. Genes shown outside the circle are transcribed clockwise, and those inside are transcribed counterclockwise. The dark grey area in the inner circle indicates the CG content of the plastome.
Compared with Amentotaxus, Pseudotaxus, Taxus and Torreya, massive genic rearrangements were identified in Cephalotaxus by the progressive Mauve alignment of plastomes (Fig. 2). These variations led to Cephalotaxus plastomes being drastically different in gene arrangement from other Taxaceae genera. Nevertheless, Cephalotaxus plastomes exhibit high levels of sequence similarity at the species level. Plastome-wide mVISTA analyses (Fig. 3) detected a total of 2782 variations within the 139 245 alignment positions, representing 2 % variation in proportion among Cephalotaxus plastomes.
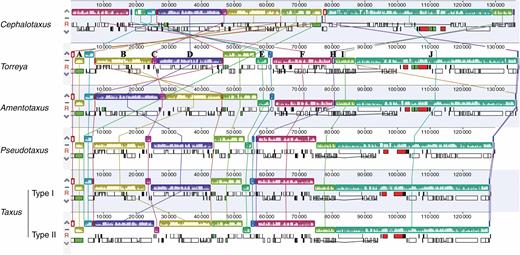
Multiple alignment resulted from Mauve showing genic arrangements detected in plastomes of Cephalotaxus and allied genera. Colour bars indicate syntenic blocks, and connecting lines indicate correspondence of blocks across plastomes.
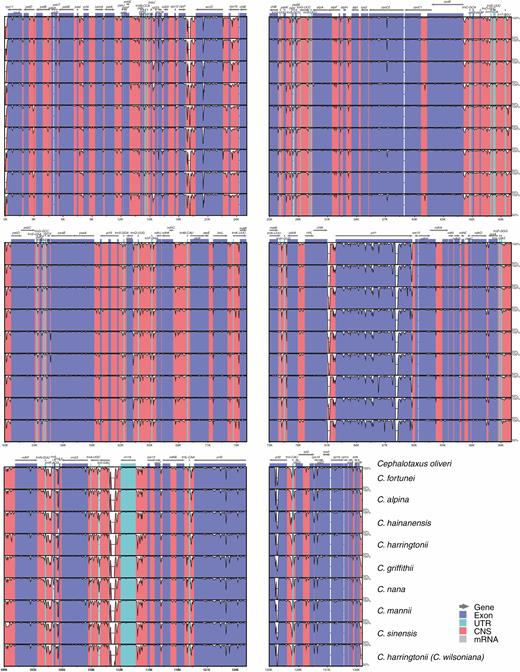
Alignment of Cephalotaxus plastomes using mVISTA, showing the percentages of sequence identity (y-axis).
Phylogenetic reconstruction
The ML and BI analyses generated highly congruent tree topologies. The monophyly of Cephalotaxus (Cephalotaxaceae) was supported with 100 % bootstrap (BS) and 1.0 PP in ML and BI phylogenies. Its sister clade (Taxaceae) consisting of Taxus, Pseudotaxus, Torreya and Amentotaxus was fully supported (BS = 100 %, PP = 1.0) as well. Our plastome phylogenomic analyses recovered well-supported relationships among the Taxaceae genera: the four genera were grouped into two well-supported branches in the phylogenetic tree (BS = 100 %, PP = 1.0), in which the sister relationship between Taxus and Pseudotaxus, as well as between Torreya and Amentotaxus, received strong support (BS = 100 %, PP = 1.0). Within Cephalotaxus, C. oliveri was the earliest diverging species and sister to the clade consisting of the remaining species. Cephalotaxus harringtonii was sister to the clade including the other species, with this clade further containing two sister clades, one including four species (C. hainanensis, C. mannii, C. fortunei and C. griffithii), and the other including three species (C. sinensis, C. alpina and C. nana). Overall, our plastome-based phylogeny satisfactorily resolved the infrageneric relationships of Cephalotaxus: except for the C. fortunei and C. griffithii clade, all nodes within the genus are robust with a BS and PP of 100 % and 1.0, respectively (Fig. 4).
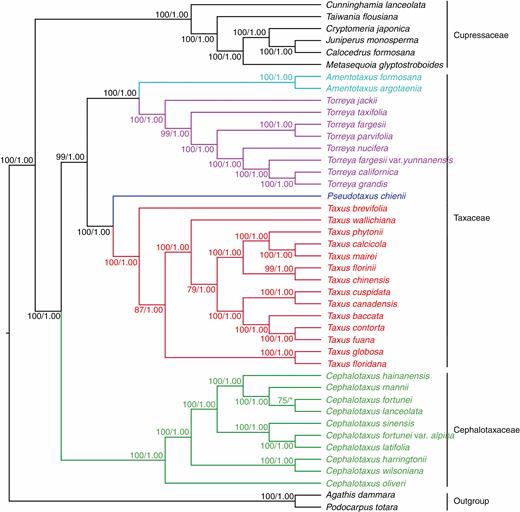
Phylogenetic relationships between Cephalotaxus and allied genera reconstructed by analyses of 81 plastid protein-coding genes using maximum likelihood (ML) and Bayesian inference (BI) methods. Numbers above branches indicated ML bootstrap percentage (BS) and BI posterior probability (PP).
Divergence time estimation
The fossil-calibrated molecular dating (Fig. 5) indicated that the diversification of extant Cephalotaxus species initiated around 20.85 Mya [95 % highest posterior density (HPD): 39.30–8.52 Mya], which is the Oligocene/Miocene transition, with C. oliveri being the earliest diverging species in the genus. Subsequently, the speciation of C. harringtonii occurred at 8.02 Mya (95 % HPD: 15.22–3.22 Mya), in the late Miocene. The diversification of the remaining species was dated to 4.68 Mya (95 % HPD: 9.03–3.22 Mya), around the Miocene/Pliocene boundary, leading to the split of the clade including C. hainanensis, C. mannii, C. fortunei and C. griffithii, and the other consisting of C. sinensis, C. alpina and C. nana. The divergence of the aforementioned terminal species occurred in the Pleistocene. Additionally, the BEAST analyses assumed a median age of 144.47 Mya (95 % HPD: 160.16–126.11 Mya) for the crown age of Taxaceae. The crown ages of Amentotaxus, Torreya, and Taxus were dated to 2.06 Mya (95 % HPD: 4.39–0.56 Mya), 9.09 Mya (95 % HPD: 16.90–4.25 Mya) and 28.75 Mya (95 % HPD: 46.05–16.43 Mya), respectively. Similar to Cephalotaxus, the most extensive species divergence events in these genera, which are responsible for the occurrence of the majority of the extant species in each genus, occurred during late Miocene to Pleistocene (Fig. 5).
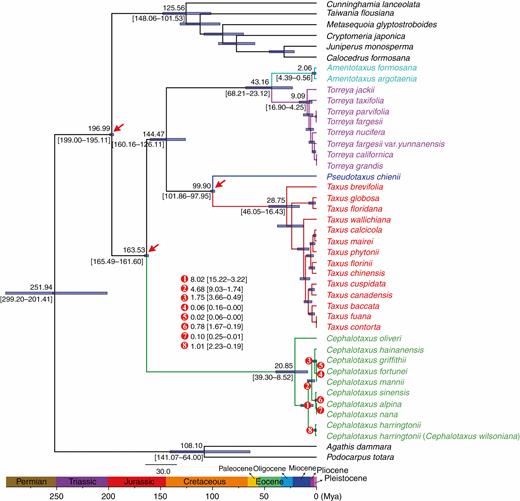
Divergence time estimation based on 81 plastid protein-coding genes. Numbers above and under the branches represent mean divergent ages and 95 % confidence interval of each node, respectively. Red arrows show the calibrating points for molecular dating. Divergence time and the timeline are indicated in million years ago (Mya).
DISCUSSION
Phylogenetic inferences and taxonomic implications
Previous studies based on single- or multilocus DNA sequence data failed to recover an unambiguous relationship between Cephalotaxus and closely related genera (Chaw et al., 1997; Cheng et al., 2000; Quinn et al., 2002; Hao et al., 2008; Lu et al., 2014). Based on a large dataset of 81 protein-coding genes that contain more variable sites and parsimoniously informative variations than have previously been available (Supplementary data Table S2), and by far the most comprehensive taxonomic sampling (all extant Cephalotaxus species were included, and Taxus and Torreya were well represented), our plastome phylogenomic analyses not only reconstructed robust relationships of Cephalotaxus to allied genera, with high support for each intergeneric node (Fig. 4), but also provided a well-supported resolution of interspecific relationships within Cephalotaxus. Our results further confirmed that phylogenetic reconstruction based on plastome sequence data can effectively resolve historical problems in phylogenetically perplexing plant groups.
Although the sister relationship between Torreya and Amentotaxus has been commonly revealed by previous studies (Chaw et al., 1997; Cheng et al., 2000; Wang and Shu, 2000; Quinn et al., 2002; Hao et al., 2008; Lu et al., 2014; Majeed et al., 2018; Ran et al., 2018; X. Zhang et al., 2019), the position of these two genera remains unresolved. For instance, the phylogenetic analyses based on 18S ribosomal DNA (Chaw et al., 1997), ribosomal ITS regions (Cheng et al., 2000; Hao et al., 2008), LFY and NLY genes (Lu et al., 2014) and RNA sequencing (Majeed et al., 2018) revealed that they are closer to Cephalotaxus (Cephalotaxaceae) than to Taxaceae. However, phylogenetic reconstruction using plastid matK (Cheng et al., 2000), a combination of plastid matK, rbcL, trnL–F and psbA–trnH (Hao et al., 2008), transcriptomic data (Ran et al., 2018) and plastomes (X. Zhang et al., 2019; this study) yielded opposite results. Notably, those studies that reconstructed phylogeny based on nuclear sequence data, i.e. Chaw et al. (1997), Cheng et al. (2000), Lu et al. (2014) and Majeed et al. (2018), had limited taxonomic sampling from Cephalotaxaceae and Taxaceae. Accordingly, phylogenetic errors or uncertainty in the tree topology resulting from limited taxon sampling seem inevitable (Rokas and Carroll, 2005; Philippe et al., 2011). Although the ribosomal ITS sequences of a wider spectrum of taxon representing Cephalotaxaceae and Taxaceae were analysed by Hao et al. (2008), neither ML nor BI phylogeny was able to provide strong support to the sister relationship between Cephalotaxus and the Torreya + Amentotaxus clade.
With a much larger taxon sampling than has previously been available, plastome phylogenomics were capable of providing valuable and credible insights for elucidating the long-standing controversies in the relationships among Amentotaxus, Cephalotaxus, Pseudotaxus, Taxus and Torreya, and thus contribute to a clear-cut delineation between Cephalotaxaceae and Taxaceae. Briefly, Cephalotaxus (Cephalotaxaceae) was resolved as sister to Taxaceae; within Taxaceae, the sister relationships between Taxus and Pseudotaxus, as well as between Torreya and Amentotaxus, were strongly supported (Fig. 5). Although the plastomes of Cephalotaxus and allied genera are paternally inherited (Mogensen, 1996) and analysis of plastome DNA sequences can only represent the paternal histories of these genera, the relationships among these genera revealed by our data are highly congruent with that of transcriptome-based phylogeny (Ran et al., 2018). This implies that the previous disagreements regarding the systematic position of Amentotaxus and Torreya most probaby result from limited taxonomic sampling (e.g. Chaw et al., 1997; Lu et al., 2014; Majeed et al., 2018) or inadequate sequence variations (Hao et al., 2008) rather than conflicts between plastid and nuclear datasets. In view of this, the plastome-based phylogeny reconstructed in this study can credibly reflect the evolutionary relationships among these genera.
The relationships revealed by our data are consistent with some morphological characteristics: the two-ovulate bracts in the seed cones distinguish Cephalotaxus from the single-ovulate bracts of the rest of the genera (Xiao et al., 2008); among the Taxaceae genera, the sister relationship between Taxus and Pseudotaxus is supported by the seeds being imperfectly enveloped by a cup-like aril, in contrast to seeds completely enclosed by aril in the sister genera Torreya and Amentotaxus (Price, 1990). Remarkably, large-scale plastome structural rearrangements were observed in Cephalotaxaceae, making it distinct from Taxaceae in the organization of plastid genes (Fig. 2). In addition to morphological differences (Xiao et al., 2008), embryological investigations also identified clear distinctions between Cephalotaxaceae and Taxaceae (Singh, 1961). Collectively, these differences convincingly justify the distinctiveness of Cephalotaxaceae and Taxaceae, and thus they should be recognized as separate families. Therefore, our results offer plastome-based phylogenomic evidence to recognize Cephalotaxaceae as a monotypic family sister to Taxaceae (Cheng et al., 2000), and support the classification proposed by Janchen (1949), who divided Taxaceae into two tribes, Torreyeae (including Amentotaxus and Torreya) and Taxeae (including Pseudotaxus and Taxus).
The robust phylogeny reconstructed in this study also provides new insights into the species delineation in Cephalotaxus (Fig. 4). For instance, the well-supported sister relationship between C. wilsoniana and C. harringtonii was recovered, providing molecular evidence to support treating C. wilsoniana as either a conspecific variety (Fu, 1999; Farjon, 2010) or a synonym of the latter species (Lang et al., 2013; J. W. Zhang et al., 2019). In addition, the tree topologies indicated that C. alpina is phylogenetically distinct from C. fortunei, supporting that it should be recognized as a separate species (Fu, 1984; Lang et al., 2013; J. W. Zhang et al., 2019) rather than a conspecific variety under C. fortunei (Fu, 1999; Farjon, 2010). Nevertheless, as shown by our results, the treatment of C. mannii as a synonym of either C. harringtonii (Lang et al., 2013) or C. hainanensis (J. W. Zhang et al., 2019), as well as reducing C. sinensis to C. harringtonii (Lang et al., 2013; J. W. Zhang et al., 2019) are likely to be arbitrary.
Notably, none of the taxonomic revisions of Cephalotaxus is fully supported by our data, suggesting that the alpha taxonomy of the genus is still not well understood. Recently, DNA barcodes have been widely used for delineating species, particularly in taxonomically difficult plant groups. Because of their low effectiveness in discriminating recently diverged taxa, the standard DNA barcodes (i.e. rbcL, psbA–trnH and ITS) yield a weak performance in identifying Cephalotaxus species (Gao et al., 2015). Our results show that analysis of the plastome DNA sequence can significantly improve phylogenetic resolution in this genus. In addition, the great potential of plastome sequencing to accurately and reliably delineate species in taxonomically challenging plant genera, such as Araucaria (Ruhsam et al., 2015), Dendrobium (Zhu et al., 2019), Panax (Ji et al., 2019b), Paris (Ji et al., 2020) and Taxus (Fu et al., 2019), has been demonstrated in recent studies. Therefore, analyses of plastome DNA sequences by sampling multiple individuals from multiple congeneric species will help future taxonomic revision of Cephalotaxus through credible species delineation.
Recent species divergence in Cephalotaxus and allied genera
The divergence of Cephalotaxus species can be attributed to climate changes in the Cenozoic, in particular the establishment of monsoonal climates in East Asia and the Pleistocene climatic fluctuations. The initiation of the Asian monsoons in the Oligocene built a connection between forests from low to high latitudes of East Asia (Sun and Wang, 2005) and a humid climate in sub-tropical areas (Wan et al., 2007; Jacques et al., 2011). From that period onward, the forest corridor would allow the ancestral lineage of extant Cephalotaxus species to retreat to sub-tropical areas in response to the Neogene global cooling (Zachos et al., 2001, 2008). Interestingly, the earliest occurrence of Cephalotaxus in sub-tropical areas is a fossil species (C. ningmingensis) found in the Oligocene Ningming Formation of Guangxi, South China, which is most similar to extant C. oliveri in morphology (Shi et al., 2010). In addition, the early divergence of Cephalotaxus, resulting in the speciation of C. oliveri, was dated to the Oligocene/Miocene boundary (Fig. 5). Collectively, it is reasonable to hypothesize that the colonization of Cephalotaxus into low latitudes of East Asia, as well as the divergence of C. oliveri, could be attributed to the combination of the Neogene global climate cooling and the onset of the Asian monsoon.
The secondary divergence event in Cephalotaxus, involving the speciation of C. harringtonii, was dated at 8.02 Mya, around the late Miocene (Fig. 5). Since then, the intensification of Asian summer monsoons established a humid climate and caused a significant expansion of forests in East Asia (Sun and Wang, 2005; Yao et al., 2011). Such climatic and environmental shifts would drive the divergence of C. harringtonii and its northward migration to Japan and the Korean Peninsula. Moreover, the Middle Miocene fossil record of Cephalotaxus from Yunnan, south-west China (J. W. Zhang et al., 2019), suggests that the enhancement of monsoonal climates in East Asia played an important role in driving the westward dispersal of Cephalotaxus as well.
It is noteworthy that the most extensive species divergence events in Cephalotaxus, resulting in the divergence of the majority of the extant species, occurred in the Pleistocene (Fig. 5). During the Pleistocene, there were at least four major glaciations in East Asia (Shi et al., 2005). The Pleistocene glaciation/interglaciation cycles, which caused dramatic contraction/expansion of species ranges in the Northern Hemisphere (Axelrod et al., 1998; Qian and Ricklefs, 2000; Petit, 2003; Qiu et al., 2011), plus the increased complexity of topography in East Asia which might have blocked the regional gene flow and boosted vicariance (Wen et al., 2014; Favre et al., 2015), are believed to have triggered rapid speciation in many plant lineages in East Asia (Li and Li, 1997; Axelrod et al., 1998; Wu et al., 2005; Lu et al., 2018). Similarly, these events would have triggered significant species radiation in Cephalotaxus.
Both fossil evidence (Florin, 1958, 1963; He and Tao, 1997; Manchester et al., 2009; Leslie, 2018) and molecular dating consistently indicate that Cephalotaxus and all Taxaceae genera are ancient plant lineages. The antiquity of these genera can also be justified by the structural divergences among their plastomes. Despite high levels of similarity in gene content, massive genic rearrangements observed at the genus level (Fig. 2) suggest that plastomes are highly divergent in gene organization. The major differences are most likely to be associated with long-term divergent evolution of plastomes among these genera and thus accumulation of mutations and structural variations as inversions and relocation of genes. Nevertheless, divergence time estimation indicates that all extant species within the genus Cephalotaxus derived from recent divergence events that initiated around the Oligocene/Miocene transition and intensified in the Pleistocene. The young ages of extant Cephalotaxus species are consistent with the low levels of DNA sequence variations among their plastomes (Fig. 3). Recent diversification of extant Cephalotaxus species implies that they are not evolutionary relicts.
We found a similar scenario of species diversification in Amentotaxus, Taxus and Torreya: most extant species of these genera also originated from recent speciation events occurring in the late Miocene, Pliocene and Pleistocene, despite the origins of these genera occurring no later than the Paleogene according to the fossil record (Florin, 1958, 1963; Manchester et al., 2009; Leslie, 2018). Recent diversification of extant species has been observed in numerous ancient gymnosperm lineages. For instance, fossil-calibrated molecular phylogenies indicate that extant cycad species are not much older than approx. 12 million years although the cycad lineage is ancient (Nagalingum et al., 2011). Recent radiations have also been reported in many coniferous lineages in East Asia, such as Cupressus (Xu et al., 2010), Juniperus (Li et al., 2012), Larix (Wei and Wang, 2004), Picea (Ran et al., 2006, 2015) and Pinus (Liu et al., 2014; Hao et al., 2015; Liu et al., 2019). The scenario of ancient lineages possessing extremely high diversity of recently diverged species suggests that these gymnosperm genera may have suffered considerable extinction during their evolutionary histories (Nagalingum et al., 2011). Therefore, occurrence of such ancient lineages in a certain area does not equate to antiquity of the extant flora, as revealed by our results and previous studies.
Conclusions
This study is by far the most comprehensive taxonomic sampling, plastome-based phylogenomic inference of Cephalotaxus. The robust phylogeny reconstructed in this study provides a perspective on the phylogenetic placement of Cephalotaxus, and contributes to a clear-cut delineation between Cephalotaxaceae and Taxaceae. The well-supported resolution of interspecific relationships within Cephalotaxus provides new evidence to clarify some of the long-standing debates in species delineation. Our data reveal that the divergence of Cephalotaxus can be attributed to the establishment of monsoonal climates in East Asia and the Pleistocene climatic fluctuations, and the species richness in this genus is associated with recent divergence events occurring in the Pleistocene. Our findings reject the idea that extant Cephalotaxus species are evolutionary relicts (Fu, 1984; Wu et al., 2005). The evolutionary profiles of Cephalotaxus provide insightful knowledge for understanding the origin and evolution of the floristic paleo-endemism in East Asia.
SUPPLEMENTARY DATA
Supplementary data are available online at https://dbpia.nl.go.kr/aob and consist of the following. Table S1: plastome sequences downloaded from GenBank. Table S2: sequence characteristics of 81 protein-coding genes involved in the phylogenetic analyses. Table S3: summary of Illumina sequencing of Cephalotaxus species. Table S4: comparison of plastome features among Cephalotaxus species. Table S5: gene content of Cephalotaxus plastomes.
ACKNOWLEDGEMENTS
We thank Drs Xun Gong and Tao Su for providing some materials for this study, and to Wenyun Chen, Qiuping Tan, Sirong Yi and Qiliang Gan for their help with field work. The authors declare no conflict of interest related to this work.
FUNDING
This work was supported by the Major Program of National Natural Science Foundation of China (grant no. 31590823), the NSFC-Joint Foundation of Yunnan Province (grant no. U1802287) and a grant from Southeast Asia Biodiversity Research Institute, Chinese Academy of Science (grant no. Y4ZK111B01).