-
PDF
- Split View
-
Views
-
Cite
Cite
Antony van der Ent, Lachlan W Casey, F Pax C Blamey, Peter M Kopittke, Time-resolved laboratory micro-X-ray fluorescence reveals silicon distribution in relation to manganese toxicity in soybean and sunflower, Annals of Botany, Volume 126, Issue 2, 1 August 2020, Pages 331–341, https://doi.org/10.1093/aob/mcaa081
- Share Icon Share
Abstract
Synchrotron- and laboratory-based micro-X-ray fluorescence (µ-XRF) is a powerful technique to quantify the distribution of elements in physically large intact samples, including live plants, at room temperature and atmospheric pressure. However, analysis of light elements with atomic number (Z) less than that of phosphorus is challenging due to the need for a vacuum, which of course is not compatible with live plant material, or the availability of a helium environment.
A new laboratory µ-XRF instrument was used to examine the effects of silicon (Si) on the manganese (Mn) status of soybean (Glycine max) and sunflower (Helianthus annuus) grown at elevated Mn in solution. The use of a helium environment allowed for highly sensitive detection of both Si and Mn to determine their distribution.
The µ-XRF analysis revealed that when Si was added to the nutrient solution, the Si also accumulated in the base of the trichomes, being co-located with the Mn and reducing the darkening of the trichomes. The addition of Si did not reduce the concentrations of Mn in accumulations despite seeming to reduce its adverse effects.
The ability to gain information on the dynamics of the metallome or ionome within living plants or excised hydrated tissues can offer valuable insights into their ecophysiology, and laboratory µ-XRF is likely to become available to more plant scientists for use in their research.
INTRODUCTION
The essentiality of manganese (Mn) for the growth of higher plants was first determined by McHargue (1922) in wheat (Triticum aestivum) and pea (Pisum sativum). Later findings established that Mn is essential for photosynthesis in a green alga (Chlorella vulgaris) (Pirson, 1994; Pirson, 1937) and in subterranean clover (Trifolium subterraneum) (Nable et al., 1984). An essential role of Mn is in water splitting and O2 evolution in photosynthesis II (PSII) (Pirson, 1937; Nable et al., 1984). Other essential roles of Mn involve respiration and as a cofactor or component of numerous enzymes (Millaleo et al., 2010; Broadley et al., 2012).
Divalent Mn2+ in the soil solution is the form absorbed by plant roots (Mulder and Gerretsen, 1952), and the concentration of which depends mostly on the quantity of numerous Mn minerals of varying solubility in the soil, on soil pH, and on soil reduction oxidation (redox) potential. A deficiency of Mn for plants occurs in soils low in Mn minerals and especially in alkaline and calcareous soils or of high redox status that often also result in Fe, Cu and Zn deficiencies (Cakmak, 2002; Schjoerring et al., 2019). In contrast, the uptake of Mn by plants increases in acid soils or those subjected to waterlogging (i.e. low redox status). The toxicity of Mn results mostly from high Mn in foliar tissues (Foy et al., 1978) although decreased root growth occurs also (Kopittke et al., 2011). Plant species differ in sensitivity to high Mn through differences in Mn uptake by roots, translocation to tops and differences in tolerance mechanisms of Mn sequestration in leaf tissues (Edwards and Asher, 1982; Horst, 1988; Blamey et al., 2015).
Liming to increase soil pH and drainage to increase soil redox status decrease Mn2+ in the soil solution, thereby decreasing Mn uptake. Added silicon (Si) may increase soil pH also (Gu et al., 2011), but many studies indicate that Si amelioration of Mn toxicity occurs largely through in planta reactions (Rogalla and Romheld, 2002; Maksimović et al., 2012). This occurs through increased apoplastic sequestration of Mn, as has been determined mostly through indirect measurement. Rogalla and Romheld (2002), for example, showed that added Si decreased Mn in apoplastic washing fluid and increased Mn bound in the cell wall. Increased apoplastic binding of Mn by Si has also been shown in other studies (Iwasaki et al., 2002; Fecht-Christoffers et al., 2006; Führs et al., 2012). More recently, synchrotron-based micro-X-ray fluorescence (µ-XRF) analysis indicated increased apoplastic accumulation of Mn with added Si in fresh, hydrated leaves of soybean (Glycine max) and sunflower (Helianthus annuus) (Blamey et al., 2018b), although these authors could only examine the distribution of Mn and not Si. Nanoscale secondary ion mass spectrometry (NanoSIMS) (Moore et al., 2014) confirmed high apoplastic Mn co-located with Si. However, NanoSIMS requires fixation prior to elemental analysis, raising concerns of experimental artefacts (Moore et al., 2014).
Synchrotron µ-XRF beamlines (such as the XFM beamline of the Australian Synchrotron, and the P06 beamline of the German Synchrotron, DESY) that are equipped with Maia and SDD detectors (Paterson et al., 2011; Schroer et al., 2016) have optimum sensitivity for transition elements with atomic number (Z) from 24 to 30 (i.e. Cr to Zn) and the metalloids As and Se (Z 33 and 34), excited with 10–23-keV monochromatic X-rays. The Maia detector can in principle detect X-rays down to 2.0 keV (hence P and S), but absorption of low-energy fluorescence X-rays in the air path causes poor sensitivity. Consequently, elements with an X-ray fluorescence energy <2 keV, such as Na, Mg, Al and Si, are beyond the reach of most synchrotron µ-XRF beamlines. There are dedicated low-energy synchrotron beamlines, such as TwinMic at the Trieste Synchrotron (Gianoncelli et al., 2016), but these facilities use an ultra-high-vacuum chamber and hence are not suitable for (fresh) hydrated plant tissues. Consequently, there are no published synchrotron µ-XRF studies of light elements (Z < P) in fresh hydrated plant tissues.
Laboratory µ-XRF has the benefit of having a vacuum-compatible sample chamber that can be purged with helium such that air-path absorption of low-energy X-rays is nearly negligible. Moreover, the use of state-of-the-art Si drift detectors (SDDs) with thin Be or polymer windows ensure high energy-resolution and limited absorption of low-energy X-rays. The X-ray sources used in instrumental µ-XRF are typically 1000–10 000 times less bright than a third-generation synchrotron X-ray source, which results in longer dwell times and possibly radiation damage. The possibility of radiation-induced damage in μ-XRF analysis (especially in fresh hydrated samples) is an important consideration that may limit the information sought from the analysis (van der Ent et al., 2018). However, no damage occurred to hydrated plant samples in a recent study using the laboratory µ-XRF facility at The University of Queensland (UQ), Australia (Harvey et al., 2020), which may be explained because the source produced a flux of 2.2 × 108 photons s−1 in a 25-μm beam spot, and at a maximum dwell of 100 ms that resulted in a deposited radiation dose of just 6.6 Gy. In a recent study, the radiation dose limit for μ-XRF analysis of hydrated plant tissues was 4.1 kGy before damage occurred (Jones et al., 2020).
The present study examined the effects of Si on foliar symptoms and Mn status of hydrated leaves of soybean and sunflower plants using laboratory µ-XRF optimized for light element analysis. A concentration of 0.5 µm Mn in solution is adequate for both these crop species but 30 µm Mn is toxic to soybean compared to an approximately 10-fold higher concentration being toxic to sunflower (Blamey et al., 2015). These species serve as a testbed for validating laboratory µ-XRF analysis of hydrated excised leaves and live plant specimens. Although it has been possible to examine Mn in living plants using synchrotron-based µ-XRF previously (Blamey et al., 2018a, b; Doolette et al., 2018), it has not been possible to simultaneously examine Si due to its low Z value. Thus, by examining the effect of Si on Mn toxicity using laboratory µ-XRF, this study aims not only to assist in understanding how Si alleviates Mn toxicity, but also to illustrate the broad utility of laboratory-based µ-XRF for examining elemental distribution in living plant tissues.
MATERIALS AND METHODS
Plant culture conditions
Three solution culture experiments studied the ameliorative effects of Si on Mn toxicity in soybean [Glycine max (L.) Merr. ‘Bunya’] and sunflower (Helianthus annuus L. ‘Ausiegold 62’) as described by Blamey et al. (2015). Briefly, four 4-d-old seedlings were transplanted into 20 L of aerated nutrient solution (pH 5.6 and ionic strength of 3 mm) with nominal concentrations of (µm): 1000 Ca, 120 NH4+-N, 95 Mg, 300 K, 10 Na, 6 Fe, 0.5 Mn, 0.5 Zn, 0.2 Cu, 1250 Cl, 670 NO3-N, 340 SO42−S, 1 B and 0.01 Mo. Soybean was grown at 5 µm P and sunflower at 20 µm P to meet this species higher P requirement. Solutions were replaced after 1 week and the Mn and Si treatments were imposed (see below). Plants were grown for a further 1 week in treatment solutions with daily addition equivalent to 1 µm P in solution to meet plant demand. Nutrient solutions remained at ~pH 5.6 through use of 15 % NH4+-N.
In Experiment 1, soybean was grown at 30 µm Mn, as MnSO4.H2O, and without or with 1.4 mm Si as Na2SiO3.9H2O. Prior to adding nutrient stock solutions and making up to 20 L, solutions with added Si were prepared by dissolving 8.18 g Na2SiO3.9H2O in 19 L deionized water and adjusting the solution pH to 5.6 with ~10 mL 1 : 1 H2O/HCl. A concentration of 30 µm Mn in 3 mm nutrient solution is toxic to soybean, an effect ameliorated by 1.4 mm Si (Blamey et al., 2018a). The same Si concentration was used by Horst et al. (1999) and Iwasaki et al. (2002) being similar to that found in soil solutions. Given its greater tolerance to high Mn, sunflower was grown at 400 µm Mn without and with 1.4 mm Si in Experiment 2. A concentration of 400 µm Mn induces Mn toxicity symptoms that are alleviated by 1.4 mm Si in solution (Blamey et al., 2018a). After 1 week of growth in treatment solutions in both experiments, plants were transferred to the UQ microXRF facility where soybean lower trifoliate leaflets and sunflower lower alternate leaves were excised prior to µ-XRF scanning. In Experiment 3, sunflower plants were grown for 2 weeks at 0.5 µm Mn without added Si before transfer to the UQ microXRF facility prior to µ-XRF scanning of opposite leaves (i.e. the oldest on the plant) of two living plants, the roots of which were in complete nutrient solution with 400 µm Mn or 400 µm Mn + 1.4 µm Si.
Laboratory µ-XRF instrumentation
The UQ microXRF facility is a custom-built system manufactured by IXRF (Austin, TX, USA). This facility consists of two 50-kV –1000-μA sources fitted with polycapillary focusing optics: an XOS microfocus Mo-target tube producing 17.4-keV X-rays (at the Mo Kα line, total flux of 2.2 × 108 photons s−1) focusing to 25 μm and an Rh-target tube producing 20.2-keV X-rays (at the Rh Kα line, total flux of 1.0 × 107 photons s−1) focusing to 5 μm. There are two KETEK SDDs of 170 mm2 collimated to 150 mm2 (with 25-μm Be-window) with CUBE preamplifiers coupled to an XIA Mercury X4 signal processing unit and an XIA FalconX (eight-channel). Energy resolution is <133 eV with a maximum input count rate of 2 × 106 s−1 (cps). The fast motion stage can address areas up to 300 × 300 mm. Dwell time per pixel is typically 50–100 ms.
Sample mounting
In Experiments 1 and 2, intact, fresh and hydrated leaves of soybean and sunflower were mounted between a sheet of 6-μm Ultralene thin film as backing and covered with 1.5 μm Etnom thin film in a tight sandwich to limit transpiration. Additionally, the leaf petiole was inserted into a small plastic envelope with nutrient solution-soaked tissue paper to ensure continuous hydration. Each scan covered an area of 20 × 4 mm and took 5 h to complete. In Experiment 3 living sunflower plants were held in place with Kapton tape, with the roots loosely wrapped in wet tissue paper soaked in nutrient solution inside a sealed 50-mL ZIP-lock bag. Leaves were covered with 6 µm Ultralene and 1.5 µm Etnom thin film apart from a 20 × 20-mm area to allow for transpiration. µ-XRF scanning was limited to a smaller area of 10 × 5 mm with each scan taking 1 h to complete and nine scans performed back-to-back. In the three experiments, the mounted sample between thin film was stretched over a Perspex frame which was magnetically attached to the x–y motion stage.
To allow accurate µ-XRF measurement of Si distribution, the instrument vacuum chamber at ~20 °C was purged with helium until the argon fluorescence peak was below the limit of detection. Thereafter, continual loss of helium from the chamber required helium purging at a rate of ~1.5 L min−1, a rate found to be adequate through monitoring of the argon peak.
Data processing
Elemental maps were generated in the Iridium software package (IXRF systems) from the sum of counts at the position of the principal peak for each element. These were each exported into ImageJ as greyscale 8-bit TIFF files, internally normalized such that each image covered the full dynamic range and displayed using ImageJ’s ‘Fire’ lookup table.
RESULTS
Visual symptoms of Mn toxicity and the effect of added Si
Small, dark spots and a mild chlorosis developed on unifoliate and lower trifoliate leaves of soybean ~2 d after exposure to 30 µm Mn. The number of small, dark spots, indicative of Mn toxicity (Wissemeier and Horst, 1992), increased over the following 5 d being especially prominent near the major veins (Fig. 1A). By this stage, the trifoliate leaves developed a distortion, known as ‘crinkle leaf’ (Heenan and Carter, 1976), with a few dark spots. The presence of 1.4 mm Si in solution with 30 µm Mn largely overcame the incidence of dark spots and the mild chlorosis (Fig. 1B). At 30 µm Mn in solution, soybean leaves attain 1000 µg g−1 Mn and 3000 µg g−1 Si without Si addition, and with 1.4 µm Si in solution this increases to 1440 µg g−1 Mn and 11 300 µg g−1 Si (Blamey et al., 2018a).
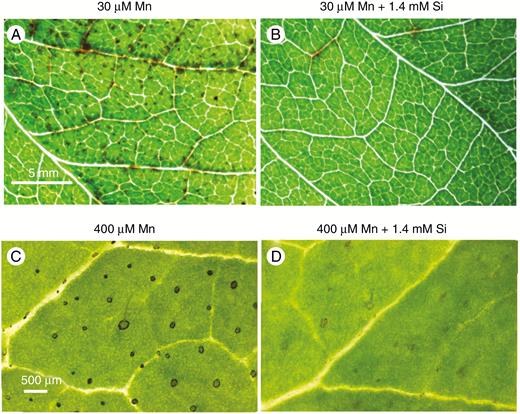
Lower trifoliate leaves of soybean grown for 7 d at 30 µm Mn (Experiment 1) developed numerous small, dark spots especially close to the major veins (A) that were largely prevented with 1.4 mm Si in solution (B). There were darkened trichomes on lower alternate leaves of sunflower (Experiment 2) grown at 400 µm Mn (C) but the darkening decreased with 1.4 mm Si in solution (D). The scale bars in A and C apply to B and D, respectively.
Sunflower grown for 7 d at 400 µm Mn developed a visible darkening at the base of trichomes on the lower stems, petioles and alternate leaves (Fig. 1C). This symptom has been identified as a tolerance mechanism through accumulation of oxidized Mn (Blamey et al., 1986; Horiguchi, 1987). Additionally, the expanding upper leaves were chlorotic, necrotic and severely distorted (Blamey et al., 2015). Added Si to the nutrient solution decreased the darkening of trichome bases on lower leaves (Fig. 1D) and decreased the Mn toxicity symptoms on the expanding upper leaves. At 400 µm Mn in solution, sunflower leaves attain 6580 µg g−1 Mn and 2900 µg g−1 Si without Si addition, and with 1.4 µm Si in solution, Mn is 6420 µg g−1 and Si 13 900 µg g−1 (Blamey et al., 2018a).
Experimental considerations
The tight thin film sandwich used to encapsulate the excised leaf samples greatly limited respiration of the tissues during the µ-XRF scanning in Experiments 1 and 2. This did not, however, result in any visible stress to the leaves (e.g. dehydration or wilting) and at the end of the experiment the leaves remained turgid (see Fig. 2, optical images). The pure helium environment used in Experiment 3 is extremely dry and resulted in markedly increased respiration. This produced a more rapid uptake and translocation of Si and Mn (within 3 h vs. 20 h) than previously observed in synchrotron µ-XRF experiments (see Blamey et al., 2018a). However, a pure (>98 %) helium environment only existed at the top ~5 cm of the instrumental chamber where the sample and X-ray source/detectors were located, and most of the whole plant (apart from a single leaf folded upward) was in a mixed air/helium environment. This meant the rest of the plant did not experience any observable dehydration or deterioration for the length of the experiment.
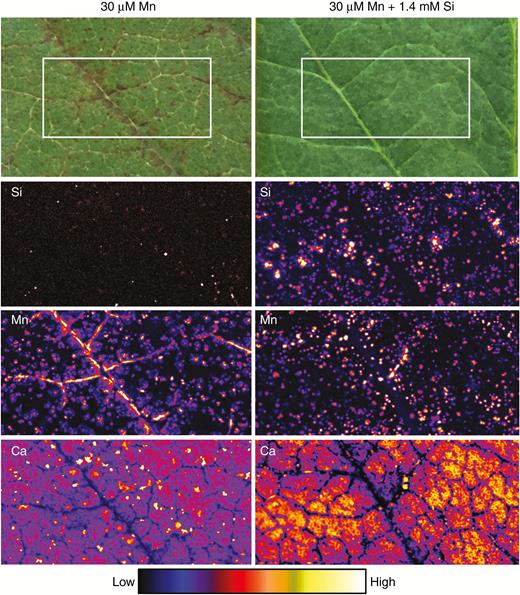
Optical images and µ-XRF scans of Si, Mn and Ca distributions in unifoliate leaves of soybean grown for 1 week in complete nutrient solutions with 30 µm Mn and 30 µm Mn + 1.4 mm Si (Experiment 1). The optical images show dark lesions, especially near the veins, caused by excess Mn that are alleviated by added Si. The µ-XRF scan is of a 10 × 5-mm area (identified by the white boxes in the optical images) acquired with a 100-ms dwell with a pixel size of 11 µm (beam size 25 µm).
Time-resolved uptake of Si and Mn examined in situ in a leaf of a living plant
We used µ-XRF analyses to obtain elemental maps from fresh/hydrated leaves of soybean and sunflower. First, for soybean exposed to 30 µm Mn without added Si, it was observed that the dark necrotic lesions near the major veins corresponded to Mn accumulations, with the distribution of dark necrotic lesions reflecting the pattern of Mn distribution (Fig. 2). As expected, concentrations of Si in the leaf in the absence of added Si were comparatively low. Upon the addition of Si, although the number of dark necrotic lesions decreased markedly, this was not associated with a more homogeneous Mn distribution within the leaf. Rather, the addition of Si to the nutrient solution actually caused the Mn to accumulate in smaller (discrete) areas to higher concentrations, although this Mn did not cause apparent visual damage to the leaf (Fig. 2). Importantly, it was noted that the high concentrations of Mn generally corresponded to areas also containing high concentrations of Si.
For sunflower, when grown in the absence of added Si, Mn tended to accumulate to high concentrations in the base of trichomes (also see the Ca distribution) (Figs 3 and 4). Unlike soybean, however, these high Mn accumulations did not cause visual damage to the leaf of sunflower, although this Mn in the base of the trichomes did cause their blackening (Fig. 1). When Si was added to the nutrient solution, the Si also accumulated in the base of the trichomes, being co-located with the Mn (Figs 3 and 4) and reducing the blackening of the trichomes (Fig. 1). Thus, like soybean, in sunflower the addition of Si did not reduce the concentrations of Mn in accumulations despite seeming to reduce its adverse effects.
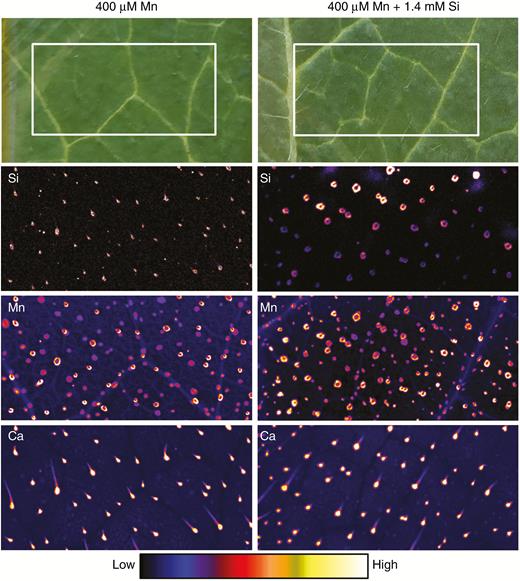
Optical images and µ-XRF scans of Si, Mn and Ca distributions in opposite (i.e. lowest) leaves of sunflower grown for 1 week in complete nutrient solutions with 400 µm Mn and 400 µm Mn + 1.4 mm Si (Experiment 2). No Mn toxicity symptoms are visible in the optical images. The µ-XRF scan is of a 10 × 5-mm area (identified by the white boxes in the optical images) acquired with a 100-ms dwell with a pixel size of 11 µm (beam size 25 µm).
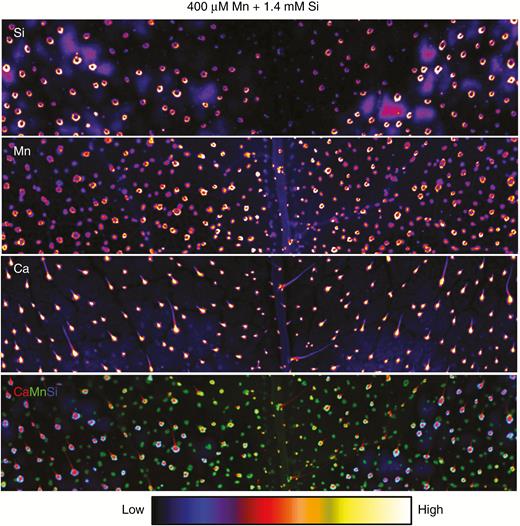
µXRF scans of Si, Mn and Ca distributions in opposite (i.e. lowest) leaves of sunflower grown for 1 week in complete nutrient solutions with 400 µm Mn + 1.4 mm Si (Experiment 2). The µ-XRF scan is of a 24 × 5-mm area acquired with a 100-ms dwell with a pixel size of 11 µm (beam size 25 µm).
Finally, we also examined living sunflower plants repeatedly over time to determine changes in the distribution of Mn and Si (Experiment 3). For Si, concentrations in the trichomes (dark blue bars) increased markedly over the 9-h experimental period whilst concentrations of Si in the remaining leaf tissue also increased but to a much lower extent (Fig. 5). For Mn, which was also supplied in the basal solution prior to commencement of the experiment, concentrations remained relatively constant during the 9-h experimental period, both within the trichomes and within the surrounding tissues (Fig. 5).
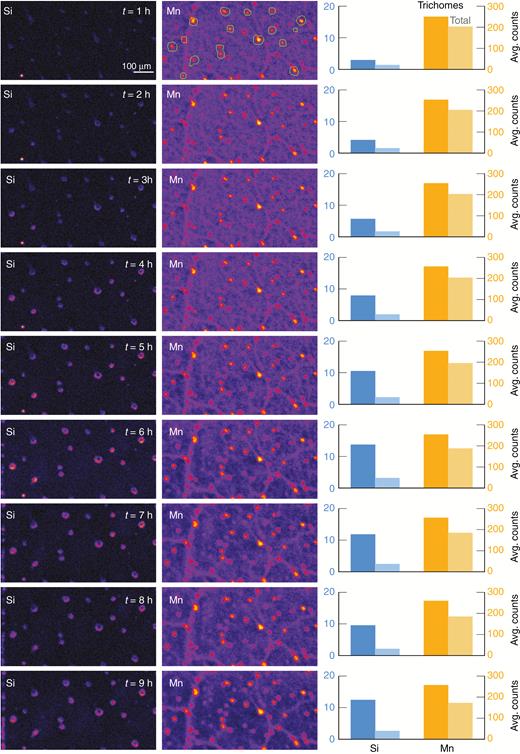
µXRF maps of Si and Mn of a fresh hydrated sunflower leaf (Experiment 3). The bar graphs on the left side show the average count per second for Si and Mn. The darker bars are signal from trichomes only (defined by the green outlines shown on the initial Mn map), and the light bars are the average across the whole image. The µ-XRF scan is of a 5.4 × 4-mm area acquired with a 50-ms dwell with a pixel size of 11 µm (beam size 25 µm).
DISCUSSION
Effect of Si on Mn toxicity
The addition of elevated levels of Mn resulted in dark necrotic lesions in soybean (Fig. 1), with these being indicative of Mn toxicity (Heenan and Carter, 1976; Horst, 1988; Wissemeier and Horst, 1992). It was found that these necrotic lesions formed due to the accumulation of excess Mn, being particularly pronounced near veins (Figs 1 and 2). These findings for soybean are consistent with those published previously – the accumulation of Mn, starting in the cell wall, causes toxic effects and results in the formation of necrotic lesions (Blamey et al., 2018a, b). In sunflower, Mn accumulated within the non-glandular trichomes, resulting in their darkening, especially around the base where the Mn presumably accumulated in the cell walls of the basal cells (Figs 1, 3 and 4). This is again consistent with previous findings, with the accumulation of Mn in trichomes being an important tolerance mechanism in sunflower (Blamey et al., 1986, 2018a).
The addition of Si prevented the formation of the dark necrotic lesions in soybean as well as prevented the darkening of the trichomes in sunflower (Fig. 1). However, despite alleviating these symptoms of Mn toxicity, this was not because Si caused a more even (homogeneous) distribution of Mn. Rather, despite the addition of Si, Mn continued to accumulate in discrete areas – being most pronounced near veins in soybean and at the base of trichomes in sunflower (Figs 1–4). Indeed, if anything, it was apparent that Si actually increased localized concentrations of Mn, with this being consistent with previous findings in these plant species (Blamey et al., 2018a). Thus, Si-induced alleviation of the toxic effects of Mn in soybean and sunflower cannot be ascribed to a more homogeneous distribution of Mn, as has been suggested previously (Williams and Vlamis, 1957; Horst and Marschner, 1978).
Unlike previous studies (e.g. Blamey et al., 2018a), we have been able not to only examine the Mn distribution but also to simultaneously determine changes in the distribution of Si within the leaf tissues through the use of the laboratory-based µ-XRF. We found that the Si was often co-located with the Mn, being at the base of the trichomes in sunflower and often in the same necrotic lesions where Mn was accumulating in soybean. This provides additional evidence to support the hypothesis that Si tends to interact with the cell wall (Currie and Perry, 2007) and that Mn then sorbs to this silicate, resulting in the co-location of Mn with Si in a non-toxic form. Indeed, this is in agreement with the hypothesis of Rogalla and Romheld (2002) that Si causes an increase in cell-wall-bound Mn. In this regard, it is known that Si covalently crosslinks the hemicellulose polysaccharides in the cell wall (He et al., 2015). This mechanism by which Si alleviates Mn toxicity differs somewhat from that of other metals, such as Al. For example, it has been reported that Si alleviates Al toxicity in Sorghum bicolor by forming Al–Si complexes in the mucigel and outer apoplast that reduce the binding of Al to the cell wall (Kopittke et al., 2017).
Although other studies have previously examined Si and Mn distribution in plant tissues, to the best of our knowledge, this is the first study to examine the distribution of Si in living, hydrated plants, including for time-resolved analyses of living plants. This is because the majority of approaches used to examine elemental distribution in plants requires the dehydration of plant tissues prior to analysis (see detailed discussion below). For example, Blamey et al. (2018a) used NanoSIMS to examine the subcellular distribution of Si (and Mn) in leaf tissues of soybean and sunflower, finding that these elements were co-localized within the cell wall. Analyses of Si (and Mn) distribution have also been conducted by other authors using dehydrated and chemically fixed tissues, including in Triticum turgidum by Keller et al. (2015) and in Cucurbita moschata by Iwasaki and Matsumura (1999). In a similar manner, Neumann and zur Nieden (2001) examined the distribution of Si (and Zn, Cu, Sn, Fe and Al) when grown on a Zn- and Cu-polluted soil, with these authors using transmission electron microscopy coupled with energy-dispersive X-ray spectroscopy (TEM-EDS) to examine elemental distribution in dehydrated, fixed, embedded tissues of Cardaminopsis halleri. However, these previous studies were conducted on chemically fixed tissues due to analytical limitations of the techniques used. Although such analyses offer supreme spatial resolution and detail on anatomical features of cells, fixation and embedding is unsuitable for investigating in situ elemental distribution in plant specimens, because it leads to washing out and redistribution of elements. Consequently, the original elemental distribution is potentially drastically changed, and the alterations vary for the various protocols (Budka et al., 2004, 2005; Kachenko et al., 2008). Thus, the technique described in the present study represents a novel approach for the examination of plant tissues, allowing analysis of light elements in living plants, thereby avoiding experimental artefacts caused by elemental redistribution during sample processing, with this being especially important for elements stored within the plant tissues in soluble forms. We have illustrated this novel approach in the present study by examining Si-induced alleviation of Mn toxicity in living hydrated plant samples.
Laboratory-based µ-XRF as a technique for analysis of living plants
Various techniques can be used to examine the in situ and in vivo distribution of elements within plant tissues, and all have attendant strengths and weaknesses (van der Ent et al., 2018). Techniques requiring ultra-high vacuum, such as conventional scanning electron microscopy with energy dispersive X-ray spectroscopy (SEM-EDS) and particle-induced X-ray emission (PIXE), introduce stringent sample processing requirements for dehydration via freeze-drying or resin embedding, and these have significant potential to introduce artefacts through elemental redistribution (Punshon et al., 2009). Cryocooling for measurement of frozen-hydrated samples is one avenue to avoid this, but availability is limited and can be challenging for the relatively large sample sizes common in studies of plant morphology (Cosio et al., 2005). Environmental SEM (E-SEM) offers the possibility of low-vacuum or even near-atmospheric measurement but raises significant challenges regarding sample size, beam damage and poor sensitivity (McGregor and Donald, 2010). Laser-ablation inductively coupled plasma mass spectroscopy (LA-ICP-MS) can in principle be used on hydrated (fresh) samples under ambient conditions, but the method is destructive due to the ablation of surface tissues and has poor sensitivity in water-rich materials (Salt et al., 2008; Persson et al., 2016). Particle-induced X-ray emission has excellent sensitivity for a very wide range of elements, but, like SEM-EDS, requires ultra-high vacuum and hence samples must be dehydrated or frozen-hydrated (van der Ent et al., 2018).
µ-XRF is a powerful technique to quantitatively analyse the distribution of elements in physically large intact samples at room temperature and atmospheric pressure. Such studies have included excised hydrated plant organs such as roots or leaves (Kopittke et al., 2011; Li et al., 2019) typically undertaken at synchrotron facilities to make use of the high-flux and energy-tunability of the X-ray source (Lombi and Susini, 2009; Lombi et al., 2011a, b). The revolutionary Maia detector array (Ryan et al., 2014, 2018; Siddons et al., 2014) has reduced the required radiation dose to the point where living plants and even time-resolved pulse-change experiments are possible with live plants (Blamey et al., 2018a, b; Doolette et al., 2018). These approaches have been extensively used in various crop plants (Kopittke et al., 2018) and hyperaccumulator plants (van der Ent et al., 2018). The technical capabilities of µ-XRF have been rapidly improving, allowing for in vivo analyses of metal(loid)s with low detection limits, excellent resolution and no theoretical restrictions on sample size (Kopittke et al., 2018). These technological advances have allowed for implementation of new approaches across the plant sciences, including functional characterization with molecular biology, examining the distribution of nutrients in human food, understanding the efficacy of foliar fertilizers, and examining the distribution of metal(loid)s in hyperaccumulator plants (Kopittke et al., 2018). Laboratory-based μ-XRF has recently been used to determine the elemental distribution in hydrated plant samples, and although this showed much promise, the dwell time of 1 s per pixel caused obvious radiation damage to the specimens investigated (Fittschen et al., 2017).
There remain only very limited studies that have examined changes in elemental distribution over time in living plants using X-ray elemental techniques at synchrotron facilities (Blamey et al., 2018b; Doolette et al., 2018). This study reports for the first time an analytical method using laboratory-based μ-XRF analysis to elucidate the time-resolved in vivo distribution of Si and Mn in leaves of living plants. We developed this method on the basis of experience with developing this approach at the XFM beamline of the Australian Synchrotron (Blamey et al., 2018a, b). The determination of concentrations of elements has moved from bulk (destructive) analysis to spatially resolved approaches to (non-destructively) obtain information of the full metallome and ionome in live plants (Punshon et al., 2009; van der Ent et al., 2018). We focused on laboratory-based μ-XRF analysis to elucidate changes in Si and Mn because much is already known about the expected elemental distribution in soybean and sunflower from previous investigations at synchrotron facilities.
The revolutionary Maia detector array installed at several synchrotron beamlines has reduced per-pixel dwell times to such an extent (typically <5 ms or even faster depending on the sensitivity required) that it has become possible to undertake µ-XRF elemental mapping on physically large samples (>10 × 10 cm), including hydrated plant samples such as whole leaves (Kopittke et al., 2011; Li et al., 2019), and even time-resolved studies on live plants dosed with Mn, for example (Blamey et al., 2018a, b; Doolette et al., 2018). Such extremely fast µ-XRF elemental mapping has been the conduit of synchrotron light sources with ultra-high-flux X-ray beams (up to 4.0 × 1011 photons s−1 at the XFM beamline of the Australian Synchrotron) coupled to the Maia detector. However, improvement in laboratory-based X-ray sources (with a flux of 2.2 × 108 photons s−1 in this study) and advances in detector electronics have closed the gap between what is possible at synchrotrons and with laboratory µ-XRF instruments. The lower flux of the latter means that per-pixel times are substantially longer (100 ms in this study), resulting in longer overall scan times, but that is less of a concern as there are not the same access restriction as at synchrotrons. As such, a scan of a 50 × 20-mm area at 25-µm resolution will take ~1 h to complete at a synchrotron or ~20 h using a laboratory-based µ-XRF instrument. There are further improvements possible on this front, however. Novel ultra-high-brightness laboratory X-ray sources, such as the FAAS (Attomap, Sigray Inc.) and Metaljet (Metaljet D2 + 70 kV, Excillium AB) sources and detectors with larger solid angle, such as the SDD array (Rococo 3, PNDetector), have the potential to lower per-pixel dwell times by an order of magnitude. Monochromatic laboratory sources (DCC X-Beam, XOS Inc.) also offer orders of magnitude higher flux and sensitivity (due to the absence of the Bremsstrahlung background) at the cost of resolution, currently ~60 µm.
Another benefit is that the laboratory µ-XRF instrument is generally available when experimental needs dictate readiness: for example, if undertaking metal dosing trials on plants and if samples are to be analysed at intervals during the experiment. Although a laboratory-based system will not replace synchrotron-based µ-XRF for a number of techniques, including X-ray absorption spectroscopy, fluorescence tomography or resolution-demanding applications, it allows researchers to combine the strengths of both facilities. Laboratory µ-XRF does have an edge for its ability to scan very large samples (up to 30 × 30 cm with the UQ system) under a range of atmospheres (air, nitrogen, helium, vacuum) to optimize light element detection. These characteristics are not unique to laboratory-based system and are not technical limitations of synchrotron facilities, but to date no synchrotron beamline has this combination of capabilities available to users. Finally, laboratory µ-XRF could be used to perform elemental mapping on large samples (e.g. whole live plants), followed by investigation of target cells (e.g. to reveal subcellular features or chemical speciation) at a synchrotron facility.
Up to now it has not been possible technically to visualize the distribution of Si in hydrated plant issues at synchrotron beamlines. This study has shown that it is entirely feasible to measure light elements down to Si in hydrated plants by using a chamber purged with helium. This study was ‘assisted’ by the fact that Si concentrates in surficial trichome basal cells, as Si fluorescence is not strongly absorbed by the cellulose matrix of plant material samples. The approach used here could be used to perform µ-XRF elemental mapping for a wide range of different elements. High-energy X-rays are able to simultaneously analyse low concentrations of a very wide range of elements in hydrated plant tissue samples. Using the Mo-source (17.4 keV) K-lines of elements with Z below Mo and optimum sensitivity for the transition metals such as Fe, Mn, Co, Ni, Zn and the metalloids As and Se are available. This opens the possibility for undertaking similar experiments as presented here on a range of crop plants, as well as hyperaccumulator plants to decipher mechanisms of uptake and translocation. If specifically targeting lighter elements (Z < S), then a Cu-source (8.04 keV) offers orders of magnitude better sensitivity.
CONCLUSION
The ability to gain information on the dynamics of the metallome or ionome within living plants or excised hydrated tissues is key to functional characterization of gene expression, stress responses and environmental interactions. The costs of laboratory µ-XRF systems are comparable to those of advanced scanning electron microscopes, and therefore likely to be in the reach for most universities. It is therefore to be expected that these facilities will become available to more plant scientists for use in their research.
FUNDING
The laboratory µ-XRF instrument has been funded through the University of Queensland Major Equipment and Infrastructure grant ‘Advanced micro-X-ray Fluorescence (μ-XRF) facility for biological, medical, materials science and geochemistry’ (UQMEI1835893).
ACKNOWLEDGEMENTS
The authors acknowledge the support of Microscopy Australia at the Centre for Microscopy and Microanalysis, at the University of Queensland.