-
PDF
- Split View
-
Views
-
Cite
Cite
Christopher R Hatcher, David B Ryves, Jonathan Millett, The function of secondary metabolites in plant carnivory, Annals of Botany, Volume 125, Issue 3, 14 February 2020, Pages 399–411, https://doi.org/10.1093/aob/mcz191
- Share Icon Share
Abstract
Carnivorous plants are an ideal model system for evaluating the role of secondary metabolites in plant ecology and evolution. Carnivory is a striking example of convergent evolution to attract, capture and digest prey for nutrients to enhance growth and reproduction and has evolved independently at least ten times. Though the roles of many traits in plant carnivory have been well studied, the role of secondary metabolites in the carnivorous habit is considerably less understood.
This review provides the first synthesis of research in which secondary plant metabolites have been demonstrated to have a functional role in plant carnivory. From these studies we identify key metabolites for plant carnivory and their functional role, and highlight biochemical similarities across taxa. From this synthesis we provide new research directions for integrating secondary metabolites into understanding of the ecology and evolution of plant carnivory.
Carnivorous plants use secondary metabolites to facilitate prey attraction, capture, digestion and assimilation. We found ~170 metabolites for which a functional role in carnivory has been demonstrated. Of these, 26 compounds are present across genera that independently evolved a carnivorous habit, suggesting convergent evolution. Some secondary metabolites have been co-opted from other processes, such as defence or pollinator attraction. Secondary metabolites in carnivorous plants provide a potentially powerful model system for exploring the role of metabolites in plant evolution. They also show promise for elucidating how the generation of novel compounds, as well as the co-option of pre-existing metabolites, provides a strategy for plants to occupy different environments.
Introduction
Secondary plant metabolites are increasingly being considered as important drivers of plant diversification and evolution (Stevenson et al., 2017). This is in part due to their involvement in a large number of fundamental processes associated with plant fitness: regulating growth and interacting with the environment, conspecifics, competitors and symbionts, and defence against abiotic and biotic stress (Hartmann, 2007; Kessler and Baldwin, 2007; Agrawal, 2011). Plants retain a high metabolic diversity and have the genetic capacity to quickly generate novel compounds due to gene duplication and divergence (Pichersky and Gang, 2000). Plants are therefore able to potentially adapt to new environments at higher rates than explained by mutation (Moore et al., 2014). If secondary plant metabolites play an important role in plant evolutionary adaptation, they should be inherently involved in processes specific to the characteristics of the plant’s ecology. The role of metabolites in adaptation may, therefore, be greater than previously considered, which would imply that we are missing important information on plant evolutionary ecology (Moore et al., 2014; Nishida, 2014). Consequently, similar patterns of metabolite production may emerge among closely and distantly related taxa that have evolved under similar environmental conditions.
Carnivorous plants are a diverse, polyphyletic group of flowering plants, generally restricted to nutrient-poor sites (Ellison and Adamec, 2018). Plant carnivory has evolved independently at least ten times in five orders (Fleischmann et al., 2018). Carnivorous plants represent an example of convergent evolution of a single syndrome (Fig. 1), to enhance growth and reproduction by attaining nutrients from prey. The benefits of carnivory are numerous: increased growth rate, earlier flowering, increased seed set, increased rate of photosynthetic output, increased nutrient uptake via roots after prey capture and increased seedling growth (Schulze et al., 2001; Adamec, 2002; Pavlovič et al., 2009; Hatcher and Hart, 2014). Within carnivorous plants, there is striking evolutionary convergence [e.g. pitcher plants; labelled (c) in Fig. 1], but also a wide diversity of morphological and physiological approaches to attract, capture, digest and assimilate prey, even within single evolutionary lineages (e.g. Nepenthales). It is now clear that, to some extent, plant carnivory has a biochemical basis (Table 1). Accordingly, carnivorous plants are an ideal study system to understand the role of secondary plant metabolites in evolutionary ecology (Thorogood et al. 2017).
Secondary plant metabolites involved in carnivory present in more than one genus. Ticks represent the 26 compounds present across independent lineages of carnivory. Ticks within boxes represent the presence of a compound found within different genera of the same carnivorous ancestor. ‘Role’ indicates a role in the carnivorous habit. Dark grey indicates Nepenthales, light grey indicates Ericales lineage of carnivory. Note that some compound isomers are identified, but in some studies this is not included. A full list and references is included in Supplementary Data Table S1
Role . | Metabolite . | Group . | Aldrovanda . | Drosophyllum . | Triphyophyllum . | Dionaea . | Drosera . | Nepenthes . | Darlingtonia . | Heliamphora . | Sarracenia . |
---|---|---|---|---|---|---|---|---|---|---|---|
Attraction | Heptadecane | Alkane | ✓ | ✓ | |||||||
Attraction | Tridecane | Alkane | ✓ | ✓ | |||||||
Attraction | Tetradecanoic acid | Alkanoic acid | ✓ | ✓ | |||||||
Attraction | Hexadecanoic acid ethyl ester | Alkanoic acid ester | ✓ | ✓ | |||||||
Attraction | Isoamyl acetate | Alkanoic acid ester | ✓ | ✓ | |||||||
Attraction | Palmitic acid methyl ester | Alkanoic acid ester | ✓ | ✓ | |||||||
Attraction | Nonanal | Alkanoic aldehyde | ✓☑ | ✓☑ | ✓ | ||||||
Attraction or digestion | Benzoic acid | Aromatic acid | ✓☑ | ✓☑ | ✓☑ | ✓ | |||||
Attraction | Benzyl acetate | Aromatic acid ester | ✓ | ✓ | |||||||
Attraction | Benzyl benzoate | Aromatic acid ester | ✓ | ✓ | |||||||
Attraction | Ethyl benzoate | Aromatic acid ester | ✓ | ✓ | |||||||
Attraction | Methyl benzoate | Aromatic acid ester | ✓ | ✓ | |||||||
Attraction | Methyl salicylate | Aromatic acid ester | ✓☑ | ✓☑ | ✓ | ||||||
Attraction | Phenylethyl acetate | Aromatic acid ester | ✓ | ✓ | |||||||
Attraction | 2-Phenylethanol | Aromatic alcohol | ✓☑ | ✓☑ | ✓ | ||||||
Attraction | Benzyl alcohol | Aromatic alcohol | ✓☑ | ✓☑ | ✓ | ||||||
Attraction | Benzaldehyde | Aromatic aldehyde | ✓☑ | ✓☑ | ✓ | ||||||
Attraction | 6-Methyl-5-hepten-2-one | Monoterpenoid | ✓☑ | ✓☑ | ✓☑ | ✓ | |||||
Attraction | Limonene | Monoterpenoid | ✓☑ | ✓☑ | ✓☑ | ✓ | |||||
Attraction | Linalool | Monoterpenoid | ✓ | ✓ | |||||||
Attraction | Myrcene | Monoterpenoid | ✓ | ✓ | |||||||
Attraction | α-Pinene | Monoterpenoid | ✓☑ | ✓☑ | ✓ | ||||||
Attraction | α-Terpinene | Monoterpenoid | ✓ | ✓ | |||||||
Attraction | p-Cymene | Monoterpenoid (aromatic) | ✓ | ✓ | |||||||
Attraction | (Z,E)-α-farnesene | Sesquiterpenoid | ✓ | ✓ | |||||||
Attraction | α-Copaene | Sesquiterpenoid | ✓ | ✓ | |||||||
Attraction | Hexadecane | Alkane | ☑ | ☑ | |||||||
Attraction | Pentadecane | Alkane | ☑ | ☑ | |||||||
Attraction | Tetradecane | Alkane | ☑ | ☑ | |||||||
Attraction | Decanal | Alkanoic aldehyde | ☑ | ☑ | |||||||
Attraction | Coniine | Alkanoic secondary amine | ☑ | ☑ | ☑ | ||||||
Attraction and capture | Erucamide | Alkenoic amide | ☑ | ☑ | ☑ | ||||||
Attraction | Acetophenone | Aromatic ketone | ☑ | ☑ | |||||||
Digestion | Myricetin | Flavanoid | ☑ | ☑ | |||||||
Attraction | Sarracenin | Monoterpene (iridoid) | ☑ | ☑ | |||||||
Digestion | 7-Methyl-juglone | Naphthoquinone | ☑ | ☑ | ☑ | ☑ | |||||
Digestion | Droserone (plumbagin derivative) | Naphthoquinone | ☑ | ☑ | |||||||
Digestion | Isoshinanolone | Naphthoquinone | ☑ | ☑ | |||||||
Capture and digestion | Plumbagin | Naphthoquinone | ☑ | ☑ | ☑ | ☑ | ☑ | ☑ | |||
Digestion | Plumbaside A | Naphthoquinone | ☑ | ☑ | |||||||
Capture/digestion/retention | Jasmonates | Oxylipin | ☑ | ☑ | ☑ | ||||||
Digestion | Gallic acid | Phenolic acid | ☑ | ☑ | ☑ | ||||||
Digestion | Protocatechuic acid | Phenolic acid | ☑ | ☑ | ☑ | ||||||
Attraction | Caryophyllene oxide | Sesquiterpene | ☑ | ☑ | |||||||
Attraction | Humulene | Sesquiterpene | ☑ | ☑ |
Role . | Metabolite . | Group . | Aldrovanda . | Drosophyllum . | Triphyophyllum . | Dionaea . | Drosera . | Nepenthes . | Darlingtonia . | Heliamphora . | Sarracenia . |
---|---|---|---|---|---|---|---|---|---|---|---|
Attraction | Heptadecane | Alkane | ✓ | ✓ | |||||||
Attraction | Tridecane | Alkane | ✓ | ✓ | |||||||
Attraction | Tetradecanoic acid | Alkanoic acid | ✓ | ✓ | |||||||
Attraction | Hexadecanoic acid ethyl ester | Alkanoic acid ester | ✓ | ✓ | |||||||
Attraction | Isoamyl acetate | Alkanoic acid ester | ✓ | ✓ | |||||||
Attraction | Palmitic acid methyl ester | Alkanoic acid ester | ✓ | ✓ | |||||||
Attraction | Nonanal | Alkanoic aldehyde | ✓☑ | ✓☑ | ✓ | ||||||
Attraction or digestion | Benzoic acid | Aromatic acid | ✓☑ | ✓☑ | ✓☑ | ✓ | |||||
Attraction | Benzyl acetate | Aromatic acid ester | ✓ | ✓ | |||||||
Attraction | Benzyl benzoate | Aromatic acid ester | ✓ | ✓ | |||||||
Attraction | Ethyl benzoate | Aromatic acid ester | ✓ | ✓ | |||||||
Attraction | Methyl benzoate | Aromatic acid ester | ✓ | ✓ | |||||||
Attraction | Methyl salicylate | Aromatic acid ester | ✓☑ | ✓☑ | ✓ | ||||||
Attraction | Phenylethyl acetate | Aromatic acid ester | ✓ | ✓ | |||||||
Attraction | 2-Phenylethanol | Aromatic alcohol | ✓☑ | ✓☑ | ✓ | ||||||
Attraction | Benzyl alcohol | Aromatic alcohol | ✓☑ | ✓☑ | ✓ | ||||||
Attraction | Benzaldehyde | Aromatic aldehyde | ✓☑ | ✓☑ | ✓ | ||||||
Attraction | 6-Methyl-5-hepten-2-one | Monoterpenoid | ✓☑ | ✓☑ | ✓☑ | ✓ | |||||
Attraction | Limonene | Monoterpenoid | ✓☑ | ✓☑ | ✓☑ | ✓ | |||||
Attraction | Linalool | Monoterpenoid | ✓ | ✓ | |||||||
Attraction | Myrcene | Monoterpenoid | ✓ | ✓ | |||||||
Attraction | α-Pinene | Monoterpenoid | ✓☑ | ✓☑ | ✓ | ||||||
Attraction | α-Terpinene | Monoterpenoid | ✓ | ✓ | |||||||
Attraction | p-Cymene | Monoterpenoid (aromatic) | ✓ | ✓ | |||||||
Attraction | (Z,E)-α-farnesene | Sesquiterpenoid | ✓ | ✓ | |||||||
Attraction | α-Copaene | Sesquiterpenoid | ✓ | ✓ | |||||||
Attraction | Hexadecane | Alkane | ☑ | ☑ | |||||||
Attraction | Pentadecane | Alkane | ☑ | ☑ | |||||||
Attraction | Tetradecane | Alkane | ☑ | ☑ | |||||||
Attraction | Decanal | Alkanoic aldehyde | ☑ | ☑ | |||||||
Attraction | Coniine | Alkanoic secondary amine | ☑ | ☑ | ☑ | ||||||
Attraction and capture | Erucamide | Alkenoic amide | ☑ | ☑ | ☑ | ||||||
Attraction | Acetophenone | Aromatic ketone | ☑ | ☑ | |||||||
Digestion | Myricetin | Flavanoid | ☑ | ☑ | |||||||
Attraction | Sarracenin | Monoterpene (iridoid) | ☑ | ☑ | |||||||
Digestion | 7-Methyl-juglone | Naphthoquinone | ☑ | ☑ | ☑ | ☑ | |||||
Digestion | Droserone (plumbagin derivative) | Naphthoquinone | ☑ | ☑ | |||||||
Digestion | Isoshinanolone | Naphthoquinone | ☑ | ☑ | |||||||
Capture and digestion | Plumbagin | Naphthoquinone | ☑ | ☑ | ☑ | ☑ | ☑ | ☑ | |||
Digestion | Plumbaside A | Naphthoquinone | ☑ | ☑ | |||||||
Capture/digestion/retention | Jasmonates | Oxylipin | ☑ | ☑ | ☑ | ||||||
Digestion | Gallic acid | Phenolic acid | ☑ | ☑ | ☑ | ||||||
Digestion | Protocatechuic acid | Phenolic acid | ☑ | ☑ | ☑ | ||||||
Attraction | Caryophyllene oxide | Sesquiterpene | ☑ | ☑ | |||||||
Attraction | Humulene | Sesquiterpene | ☑ | ☑ |
Secondary plant metabolites involved in carnivory present in more than one genus. Ticks represent the 26 compounds present across independent lineages of carnivory. Ticks within boxes represent the presence of a compound found within different genera of the same carnivorous ancestor. ‘Role’ indicates a role in the carnivorous habit. Dark grey indicates Nepenthales, light grey indicates Ericales lineage of carnivory. Note that some compound isomers are identified, but in some studies this is not included. A full list and references is included in Supplementary Data Table S1
Role . | Metabolite . | Group . | Aldrovanda . | Drosophyllum . | Triphyophyllum . | Dionaea . | Drosera . | Nepenthes . | Darlingtonia . | Heliamphora . | Sarracenia . |
---|---|---|---|---|---|---|---|---|---|---|---|
Attraction | Heptadecane | Alkane | ✓ | ✓ | |||||||
Attraction | Tridecane | Alkane | ✓ | ✓ | |||||||
Attraction | Tetradecanoic acid | Alkanoic acid | ✓ | ✓ | |||||||
Attraction | Hexadecanoic acid ethyl ester | Alkanoic acid ester | ✓ | ✓ | |||||||
Attraction | Isoamyl acetate | Alkanoic acid ester | ✓ | ✓ | |||||||
Attraction | Palmitic acid methyl ester | Alkanoic acid ester | ✓ | ✓ | |||||||
Attraction | Nonanal | Alkanoic aldehyde | ✓☑ | ✓☑ | ✓ | ||||||
Attraction or digestion | Benzoic acid | Aromatic acid | ✓☑ | ✓☑ | ✓☑ | ✓ | |||||
Attraction | Benzyl acetate | Aromatic acid ester | ✓ | ✓ | |||||||
Attraction | Benzyl benzoate | Aromatic acid ester | ✓ | ✓ | |||||||
Attraction | Ethyl benzoate | Aromatic acid ester | ✓ | ✓ | |||||||
Attraction | Methyl benzoate | Aromatic acid ester | ✓ | ✓ | |||||||
Attraction | Methyl salicylate | Aromatic acid ester | ✓☑ | ✓☑ | ✓ | ||||||
Attraction | Phenylethyl acetate | Aromatic acid ester | ✓ | ✓ | |||||||
Attraction | 2-Phenylethanol | Aromatic alcohol | ✓☑ | ✓☑ | ✓ | ||||||
Attraction | Benzyl alcohol | Aromatic alcohol | ✓☑ | ✓☑ | ✓ | ||||||
Attraction | Benzaldehyde | Aromatic aldehyde | ✓☑ | ✓☑ | ✓ | ||||||
Attraction | 6-Methyl-5-hepten-2-one | Monoterpenoid | ✓☑ | ✓☑ | ✓☑ | ✓ | |||||
Attraction | Limonene | Monoterpenoid | ✓☑ | ✓☑ | ✓☑ | ✓ | |||||
Attraction | Linalool | Monoterpenoid | ✓ | ✓ | |||||||
Attraction | Myrcene | Monoterpenoid | ✓ | ✓ | |||||||
Attraction | α-Pinene | Monoterpenoid | ✓☑ | ✓☑ | ✓ | ||||||
Attraction | α-Terpinene | Monoterpenoid | ✓ | ✓ | |||||||
Attraction | p-Cymene | Monoterpenoid (aromatic) | ✓ | ✓ | |||||||
Attraction | (Z,E)-α-farnesene | Sesquiterpenoid | ✓ | ✓ | |||||||
Attraction | α-Copaene | Sesquiterpenoid | ✓ | ✓ | |||||||
Attraction | Hexadecane | Alkane | ☑ | ☑ | |||||||
Attraction | Pentadecane | Alkane | ☑ | ☑ | |||||||
Attraction | Tetradecane | Alkane | ☑ | ☑ | |||||||
Attraction | Decanal | Alkanoic aldehyde | ☑ | ☑ | |||||||
Attraction | Coniine | Alkanoic secondary amine | ☑ | ☑ | ☑ | ||||||
Attraction and capture | Erucamide | Alkenoic amide | ☑ | ☑ | ☑ | ||||||
Attraction | Acetophenone | Aromatic ketone | ☑ | ☑ | |||||||
Digestion | Myricetin | Flavanoid | ☑ | ☑ | |||||||
Attraction | Sarracenin | Monoterpene (iridoid) | ☑ | ☑ | |||||||
Digestion | 7-Methyl-juglone | Naphthoquinone | ☑ | ☑ | ☑ | ☑ | |||||
Digestion | Droserone (plumbagin derivative) | Naphthoquinone | ☑ | ☑ | |||||||
Digestion | Isoshinanolone | Naphthoquinone | ☑ | ☑ | |||||||
Capture and digestion | Plumbagin | Naphthoquinone | ☑ | ☑ | ☑ | ☑ | ☑ | ☑ | |||
Digestion | Plumbaside A | Naphthoquinone | ☑ | ☑ | |||||||
Capture/digestion/retention | Jasmonates | Oxylipin | ☑ | ☑ | ☑ | ||||||
Digestion | Gallic acid | Phenolic acid | ☑ | ☑ | ☑ | ||||||
Digestion | Protocatechuic acid | Phenolic acid | ☑ | ☑ | ☑ | ||||||
Attraction | Caryophyllene oxide | Sesquiterpene | ☑ | ☑ | |||||||
Attraction | Humulene | Sesquiterpene | ☑ | ☑ |
Role . | Metabolite . | Group . | Aldrovanda . | Drosophyllum . | Triphyophyllum . | Dionaea . | Drosera . | Nepenthes . | Darlingtonia . | Heliamphora . | Sarracenia . |
---|---|---|---|---|---|---|---|---|---|---|---|
Attraction | Heptadecane | Alkane | ✓ | ✓ | |||||||
Attraction | Tridecane | Alkane | ✓ | ✓ | |||||||
Attraction | Tetradecanoic acid | Alkanoic acid | ✓ | ✓ | |||||||
Attraction | Hexadecanoic acid ethyl ester | Alkanoic acid ester | ✓ | ✓ | |||||||
Attraction | Isoamyl acetate | Alkanoic acid ester | ✓ | ✓ | |||||||
Attraction | Palmitic acid methyl ester | Alkanoic acid ester | ✓ | ✓ | |||||||
Attraction | Nonanal | Alkanoic aldehyde | ✓☑ | ✓☑ | ✓ | ||||||
Attraction or digestion | Benzoic acid | Aromatic acid | ✓☑ | ✓☑ | ✓☑ | ✓ | |||||
Attraction | Benzyl acetate | Aromatic acid ester | ✓ | ✓ | |||||||
Attraction | Benzyl benzoate | Aromatic acid ester | ✓ | ✓ | |||||||
Attraction | Ethyl benzoate | Aromatic acid ester | ✓ | ✓ | |||||||
Attraction | Methyl benzoate | Aromatic acid ester | ✓ | ✓ | |||||||
Attraction | Methyl salicylate | Aromatic acid ester | ✓☑ | ✓☑ | ✓ | ||||||
Attraction | Phenylethyl acetate | Aromatic acid ester | ✓ | ✓ | |||||||
Attraction | 2-Phenylethanol | Aromatic alcohol | ✓☑ | ✓☑ | ✓ | ||||||
Attraction | Benzyl alcohol | Aromatic alcohol | ✓☑ | ✓☑ | ✓ | ||||||
Attraction | Benzaldehyde | Aromatic aldehyde | ✓☑ | ✓☑ | ✓ | ||||||
Attraction | 6-Methyl-5-hepten-2-one | Monoterpenoid | ✓☑ | ✓☑ | ✓☑ | ✓ | |||||
Attraction | Limonene | Monoterpenoid | ✓☑ | ✓☑ | ✓☑ | ✓ | |||||
Attraction | Linalool | Monoterpenoid | ✓ | ✓ | |||||||
Attraction | Myrcene | Monoterpenoid | ✓ | ✓ | |||||||
Attraction | α-Pinene | Monoterpenoid | ✓☑ | ✓☑ | ✓ | ||||||
Attraction | α-Terpinene | Monoterpenoid | ✓ | ✓ | |||||||
Attraction | p-Cymene | Monoterpenoid (aromatic) | ✓ | ✓ | |||||||
Attraction | (Z,E)-α-farnesene | Sesquiterpenoid | ✓ | ✓ | |||||||
Attraction | α-Copaene | Sesquiterpenoid | ✓ | ✓ | |||||||
Attraction | Hexadecane | Alkane | ☑ | ☑ | |||||||
Attraction | Pentadecane | Alkane | ☑ | ☑ | |||||||
Attraction | Tetradecane | Alkane | ☑ | ☑ | |||||||
Attraction | Decanal | Alkanoic aldehyde | ☑ | ☑ | |||||||
Attraction | Coniine | Alkanoic secondary amine | ☑ | ☑ | ☑ | ||||||
Attraction and capture | Erucamide | Alkenoic amide | ☑ | ☑ | ☑ | ||||||
Attraction | Acetophenone | Aromatic ketone | ☑ | ☑ | |||||||
Digestion | Myricetin | Flavanoid | ☑ | ☑ | |||||||
Attraction | Sarracenin | Monoterpene (iridoid) | ☑ | ☑ | |||||||
Digestion | 7-Methyl-juglone | Naphthoquinone | ☑ | ☑ | ☑ | ☑ | |||||
Digestion | Droserone (plumbagin derivative) | Naphthoquinone | ☑ | ☑ | |||||||
Digestion | Isoshinanolone | Naphthoquinone | ☑ | ☑ | |||||||
Capture and digestion | Plumbagin | Naphthoquinone | ☑ | ☑ | ☑ | ☑ | ☑ | ☑ | |||
Digestion | Plumbaside A | Naphthoquinone | ☑ | ☑ | |||||||
Capture/digestion/retention | Jasmonates | Oxylipin | ☑ | ☑ | ☑ | ||||||
Digestion | Gallic acid | Phenolic acid | ☑ | ☑ | ☑ | ||||||
Digestion | Protocatechuic acid | Phenolic acid | ☑ | ☑ | ☑ | ||||||
Attraction | Caryophyllene oxide | Sesquiterpene | ☑ | ☑ | |||||||
Attraction | Humulene | Sesquiterpene | ☑ | ☑ |
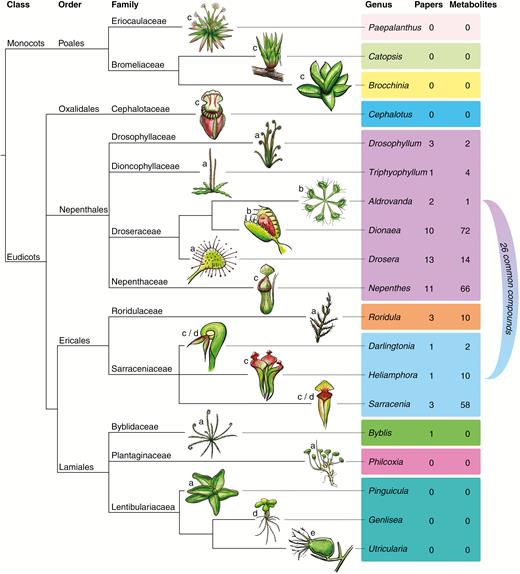
Phylogenetic tree of carnivorous plants and research and metabolites identified with known function in carnivory. Branches are to emphasize the location of carnivorous taxa and do not signify time since divergence or a metric of relatedness. Trap morphology is grouped into: (a) flypaper; (b) snap-trap; (c) pitfall; (d) lobster pot; and (e) suction bladder. Colour bands signify a common carnivorous ancestor. Tree generated using NCBI taxonomy browser, iTOL and APG (Chase et al., 2016; Letunic and Bork, 2016). Nepenthales is sensu stricto sister to Caryophyllales (Fleischmann et al., 2018). Twenty-six common compounds are found in the Nepenthales lineage and Sarraceniaceae lineage. Note that Aldrovanda and Utricularia are genera of aquatic carnivorous plants.
Determining the function of secondary metabolites in the carnivorous habit is considerably more challenging and less well understood than determining morphological function (Ellison and Adamec, 2018). This lack of knowledge is critical because growing understanding of the importance of the role of metabolites in rapid plant diversification and adaptation means that we may be missing important insight into the evolution of plant carnivory (Moore et al., 2014). Advanced analytical capabilities and reduced costs have resulted in an increase in the number of studies identifying secondary metabolites in carnivorous plants. The result is a growing body of literature describing secondary metabolites, mostly in relation to their anthropogenic use (Banasiuk et al., 2012; Egan and Van Der Kooy, 2013). We lack, however, a synthesis of current knowledge of specific biochemical function in relation to the tissue in which these compounds are produced; this is required to begin to link gene expression to secondary metabolite synthesis, carnivorous plant ecology and evolution.
In this review we synthesize current understanding of the secondary plant metabolites that are involved in the carnivorous syndrome. The aim is to use this synthesis to explore the extent to which secondary plant metabolites play a role in all aspects of plant carnivory across independent lineages. It will also highlight comparisons of secondary plant metabolite function across lineages, providing insight on the biochemical evolutionary convergence and divergence of carnivorous plants. Secondary metabolites were identified through a systematic search of Google Scholar and Web of Science using search terms ‘metabolite’, ‘carnivorous plant’ and their synonyms, carnivorous plant genera and prominent study species. We also searched the reference lists of relevant journal articles in case papers were not identified from the previous search terms. We did not include studies where the focus was on extracting secondary metabolites, rather than considering their role in carnivory. Because we are interested in the role of metabolite function in plant carnivory, we have collated metabolites under the four key stages of the carnivorous habit: prey attraction, capture, digestion, and assimilation of nutrients.
SECONDARY METABOLITES INVOLVED IN THE ATTRACTION OF PREY
Darwin (1875) was the first to suggest that carnivorous plants might attract their prey through the production of odour. It has been demonstrated that some (but by no means all) carnivorous plant species actively attract prey by mimicking fruit and flowers using olfactory or visual cues, or through the production of extrafloral nectar (Bennett and Ellison, 2009; Kreuzwieser et al., 2014). Attraction mechanisms are still, however, relatively poorly understood or inconsistent across species and the evidence is, in some cases, controversial.
Olfactory cues
Volatile organic compounds (VOCs), compounds volatile at ambient temperature, are involved in the attraction of prey; such animal attraction is not uncommon elsewhere in nature (Pichersky and Gershenzon, 2002). These compounds can increase the frequency of prey interaction but have also been demonstrated to enable carnivorous plants to select prey by species and potentially by size (Di Giusto et al., 2010; Kreuzwieser et al., 2014; Hotti et al., 2017). The VOCs are by far the most researched group of metabolites produced by carnivorous plants (over half of the ~170 compounds listed in Supplementary Data Table S1). Nepenthes rafflesiana, a vine species of pitcher plant, synthesizes at least 54 different VOCs that, as a blend of compounds, attract their prey (Di Giusto et al., 2010). Kreuzwieser et al. (2014) identified more than 60 different VOCs released by Dionaea muscipula (Venus flytrap). Their comparison of VOC concentrations before and after prey capture suggested that 20 compounds were directly related to prey attraction, through mimicry of fruit and flowering scents. They also found differences in levels of 1-(1-cyclohexen-1-yl)-ethanone and dodecanoic acid, propyl ester before and after prey capture. These compounds have not previously been attributed as attractants to insects, suggesting that D. muscipula produces a mix of VOCs, some of which are the same as those in flowers and fruit, whilst others are novel. The synthesis of fruit- and flower-mimicking VOCs alongside novel VOCs may be a strategy for avoiding pollinator–prey conflict (El-Sayed et al., 2016). Alternatively, it might be the case that novel VOCs were part of the evolution of snap-traps in D. muscipula as an adaptation for the capture of larger prey than co-occurring carnivorous plants (Hutchens and Luken, 2009).
Prey spectrum specialization through the use of modified secondary plant metabolites is evident in other carnivorous plant genera. Nonanal is a floral scent compound, the synthesis of which is widespread in plants. This VOC is an attractant for Nematocera species, which are abundant in the natural habitats of carnivorous plant species (i.e. bogs) and are common prey taxa (Ellison and Adamec, 2018). This VOC is synthesized by the closely related carnivorous plants Sarracenia spp. and Darlingtonia californica and confirmed to be a prey attractant through Y-tube olfactory choice assays ex situ (Di Giusto et al., 2010; Hotti et al., 2017). In the pitcher plant, N. rafflesiana, synthesis of VOCs differs between upper and lower pitchers; this attracts a different spectrum of prey – aerial insects to upper pitchers and ants to lower pitchers (Di Giusto et al., 2010). In addition, juvenile or freshly opened N. rafflesiana pitchers that have captured few or no prey emit flower-like scents (Bauer et al., 2009); putrefaction odours are produced by older pitchers that have accumulated prey. It is not clear whether this is through compounds emitted by the leaf tissue, or is a function of actual prey decomposition (Jürgens et al., 2009). This demonstrates the potential for prey spectrum specialization within different tissues of a single individual, controlled by secondary metabolite synthesis. These results also highlight how the main biochemical drivers of attraction may change throughout the life of a plant or leaf.
There are several instances in which a VOC linked to the attraction of organisms may also serve an additional function in plant carnivory. Coniine is a similar metabolite to floral scent compounds found in non-carnivorous plants (Roberts and Wink, 1998). This compound has been found to be produced in the lids and pitchers of Sarracenia spp., suggesting a prey attraction function (Hotti et al., 2017). In addition, coniine may play a role in retaining prey if concentrations are sufficient as it can act as an insect-stunning agent, though actual concentrations required for this to be effective have not been found in situ. Pulegone, a floral scent compound also found in Sarracenia spp., functions as an attractant but may also function as an insecticide (Franzios et al., 1997), demonstrating the possibility that it plays a role in the killing of prey as well as attraction. Tetradecanoic acid and hexadecanoic acid methyl ester are both floral scent compounds and have also been found in the traps of D. muscipula and Sarracenia spp. (Kreuzwieser et al., 2014). Hexadecanoic acid production is increased by D. muscipula after feeding, suggesting some carnivorous function. Hexadecanoic acid is a major component of oils in plants and may act as a surfactant or be involved in enzymatic activity during prey digestion, though this has not been tested.
Production of insect reproductive pheromones for attraction of pollinators is well established in nature (Nishida, 2014). Some VOCs produced by carnivorous plant species are also thought to attract prey by mimicking insect reproductive pheromones. One individual of Sarracenia flava was found to contain 14-β-pregna, a VOC known to be a sex pheromone of the insect Eurygaster maura, though whether the plant produced this cannot be confirmed (and hence this is not included in Supplementary Data Table S1; see Hotti et al., 2017). In addition, Sarracenia spp. also produce actinidine and trans-jasmone, pheromones used by species of Hymenoptera. The direct role of these VOCs for prey attraction has not been tested under a single-compound regime, though a blend of VOCs is likely to enhance prey attraction efficiency similar to floral blends (Kreuzwieser et al., 2014).
This co-option of existing VOCs – from fruit and floral compounds for use as a prey attractant – demonstrates the versatility of secondary metabolites to provide multiple functions, differentiated by the location or tissue from which the compound is emitted. The potential independent evolution of VOCs similar to those used by insects to attract other insects is an example of convergent evolution driven by evolutionary pressure to attract insects, though for entirely different purposes. Plants’ deception of insects is well documented, but carnivorous plants have adopted a multitude of strategies to lure and deceive animals for the ultimate goal of nutrient acquisition, in addition to reproduction (Ellison and Adamec, 2018).
Further work is needed to identify the specific roles of these novel and pre-existing compounds and to determine whether their effectiveness is dependent on the compounds acting as a blend, or whether each compound acts as an effective attractant to prey. To date, no studies have investigated how VOC blends may change over time or growth stages, i.e. longer than immediately before and after prey capture. Dionaea muscipula, for example, captures an entirely different spectrum of prey as seedlings compared with mature stages (Hatcher and Hart, 2014); it seems possible that their volatile blend may change over the life cycle of the plant to reflect this specialization. A research gap exists in understanding how plastic the metabolic profile of carnivorous plants is in response to growth stage, nutrient status and environmental stimuli.
Visual cues
Secondary metabolites constitute the pigments that plants use to create the visual cues that play a key role in plant–animal communication. Anthocyanins, for example, produce a red colour that advertises fruit ripeness. Anthocyanins, flavonoids, carotenoids and betalains are responsible for flower colour and pattern, which attract pollen vectors for reproduction and dispersal (Tanaka et al., 2008). These pigments have been assumed to attract prey to carnivorous plants, due to the distinctive red trap colouration of many carnivorous plants (Fig. 1). The anthocyanins and co-pigments that constitute this colouration in trap leaves include kaempferol, quercetin and cyanidin (Sheridan and Griesbach, 2001). A variety of carnivorous plant traps also display UV reflectance patterns. These patterns are also suggested to have a prey attraction function, and are attributed to the accumulation of phenolics, quinones and sugars (Kurup et al., 2013) (Fig. 2b). There is, however, as yet no robust evidence that pigments in traps serve a prey attraction function ubiquitously (Bennett and Ellison, 2009). Red pigmentation is not likely to be an attractant to many invertebrate species as they cannot perceive red wavelengths of light, and in some carnivorous plants red pigmentation has been shown not to impact prey capture rate (Foot et al., 2014). The potential for pigmentation as a prey attractant may be plant species–prey specific, or colouration may indirectly attract prey due to a contrast with background colouration for insect species that do not perceive light in the red frequencies (Fig. 2C). Other functions for trap pigments have been suggested, such as photoprotection or light stress, as seen in Drosera rotundifolia (Fig. 2A) (Millett et al., 2018) and nutrient stress as observed in Dionaea muscipula and Drosera spathulata (Ichiishi et al., 1999). Analysis of pigments in carnivorous plants has, however, been useful in determining phylogeny. The Venus flytrap (Dionaea muscipula), formerly grouped under Caryophyllales, synthesizes anthocyanins rather than betalains (characteristic of the majority of Caryophyllales) and this provides further support for grouping these plants under Nepenthales using molecular phylogenetic data (Ellison and Adamec, 2018). Interestingly, pigment concentrations were shown to be significantly different in petioles compared with traps of D. muscipula. In particular, cyanidin was present in the digestive glands, but absent from the non-carnivorous leaf tissue (Henarejos-Escudero et al., 2018). Given the limitations of current approaches, further work is required to determine the adaptive carnivorous function, if any, of the synthesis of these pigment metabolites.
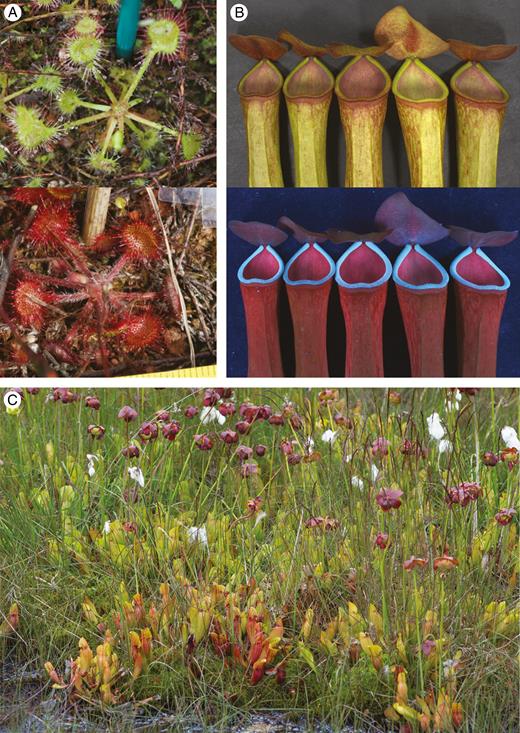
Function of secondary metabolites in trap coloration requires further investigation. (A) Drosera rotundifolia changes in colour from accumulation of anthocyanin (red pigmentation) in response to environmental stimuli, but this is not confirmed to be related to carnivory. (B) (Upper panel) Nepenthes species show redder locations, possibly to contrast the trap peristome against the background for prey attraction. (Lower panel) Nepenthes spp. also use UV florescence for prey attraction. (C) Sarracenia purpurea, with high diversity of red and green pigmentation from secondary compounds with high background contrast in situ. (Photographs of Nepenthes courtesy of Dr S. Baby in Kurup et al., 2013; Drosera rotundifolia and Sarracenia purpurea photographs are by C. R. Hatcher).
Extrafloral nectaries
Nectar is a saccharide-rich liquid produced by plants in glands or nectaries and is composed of primary metabolites including sucrose, fructose and glucose, but is also often a combination of many secondary compounds (Stevenson et al., 2017). Flowering plants possess nectaries that function as a reward or bribe to attract pollinating insects (Roy et al., 2017). Many plant species also possess extrafloral nectaries that perform functions other than pollinator attraction, such as recruiting animals that deter herbivores, and the nectar often contains additional metabolites (Marazzi et al., 2013). Extrafloral nectaries are present on the peristome of carnivorous pitfall traps in the genera Darlingtonia, Heliamphora, Sarracenia, Cephalotus and Nepenthes, and on the trap lobes of the snap-trap of D. muscipula (Płachno, 2007) (Fig. 3). Nepenthes are also known to have coprophagous relationships with birds and small mammals that perch on the pitcher rim to feed on the nectar (Clarke et al., 2009; Chin et al., 2010; Grafe et al., 2011). The chemical constituents of the nectar produced by these extrafloral nectaries have not been closely studied in carnivorous plants, though such nectaries have evolved independently at least three times in carnivorous plants and are likely to be a combination of primary metabolites (sugars) and secondary compounds such as VOCs (Fig. 3) (Givnish, 2015). It would be valuable to study the degree to which this convergent evolution also applies to nectar chemistry in order to explain how carnivorous plants may alleviate the overlap between pollinators and prey. Currently, as far as we are aware there has been no study comparing the metabolite blend of a species’ floral, extrafloral and trap-related extrafloral nectaries.
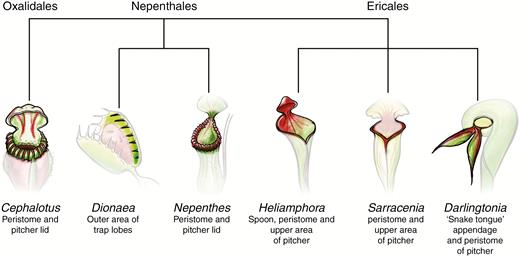
Evolution and production zones of metabolites for attraction of prey via use of extrafloral nectaries. Highlighted areas indicate the highest density of extrafloral nectaries for attraction of prey. Areas for secretion of secondary metabolites and nectar are important for prey attraction and capture. Length of phylogenetic branches does not signify relatedness or any other metric of evolution.
Presence of metabolites across independent lineages of plant carnivory for prey attraction
This review has identified 26 secondary plant metabolites involved in the attraction of prey that are present across independent lineages of carnivory. These secondary metabolites are the only known aspect of carnivory where the function of the same compound has been demonstrated across independent lineages of plant carnivory, with the exception of benzoic acid, the function of which is not confirmed but is suggested to concern attraction and digestion (Supplementary Data Table S1). There is also high overlap within different genera of the same carnivorous lineage (boxed ticks in Table 1 and light grey bars in Supplementary Data Table S1). These secondary metabolites are all VOCs and provide evidence of convergent evolution of carnivory at a biochemical scale.
The majority of these VOCs are common across non-carnivorous plant taxa as fruit or floral scents to attract pollinators. There are only a handful of carnivorous genera, however, that have been studied for the presence of VOCs for prey attraction. The majority of pre-attraction VOCs have been identified in a few studies on Nepenthes spp., D. muscipula and the separate carnivorous lineage of Sarracenia spp. Clearly, VOCs as prey attractants are the ideal focus for understanding convergent evolution of carnivory at a biochemical scale. Future work on the role of secondary metabolites in attraction should focus on determining whether other lineages of carnivorous plants attract prey through the use of VOCs, particularly VOCs already known in other species. Given that most of the compounds found to be used in the attraction of prey are also widespread as fruit or floral scents, determining whether these compounds were present before evolutionary divergence or afterwards would show the frequency of exaptation of current plant metabolites or generation of novel compounds for the carnivorous syndrome.
SECONDARY METABOLITES INVOLVED IN THE CAPTURE OF PREY
Prey capture mechanisms are the most studied aspect of carnivory in plants (Ellison and Adamec, 2018). The different approaches to prey capture result in novel and unusual morphologies and plant movements. In carnivorous plants, five distinct trapping mechanisms have been identified: (1) flypaper – leaves that secrete a sticky mucilage; (2) snap-traps – some of the most rapid plant movements in the kingdom; (3) pitchers/pitfall traps; (4) lobster pot traps, which force prey movement in one direction away from the trap entrance using downward-facing hairs; and (5) suction traps/bladder traps (Fig. 1). These trapping mechanisms rely on distinct morphological adaptation, but in many cases they also require the use of secondary metabolites to enhance capture efficiency and prey retention. Secondary metabolites can be split into two functional groups for prey capture: those that play a physical role and those that signal prey capture.
Physical trapping mechanisms
The physical role of metabolites for trapping prey includes adhesive properties as found, for example, in mucilage of Drosera spp., glue of Roridula species and the fluids of pitcher plants, some of which have viscoelastic properties, or wax crystals and metabolites in nectar within the pitcher plants relying on slippery surfaces for capture (Riedel et al., 2003; Płachno, 2007; Bauer et al., 2008). One example of how secondary metabolites are involved in these functions is the use of extrafloral nectar to decrease friction on the peristome of Nepenthes spp. pitchers (Fig. 3; Bauer et al., 2008). This increases prey capture efficiency by reducing the ability of prey (primarily ants, Formicidae) to adhere to the pitcher lip, increasing the likelihood of falling into the pitcher trap. Secondary metabolites specifically implicated in this process are as yet unknown, but given the novel, mechanical function of this nectar, chemical composition may differ from nectar produced specifically for attraction. A similar functional role is performed by erucamide in the pitcher plants of the unrelated genus Heliamphora, where it acts as a lubricating agent in the nectar produced around the peristome of the traps to increase trapping efficiency (Płachno, 2007). Plumbagin may also play a key role in prey capture for Nepenthes khasiana. Plumbagin is found on the rim of species of Nepenthes pitcher plants and is suggested to work in tandem with the slippery surface by inflicting an anaesthetic or toxic influence on prey, inhibiting their escape responses (Raj et al., 2011). Plumbagin is a secondary metabolite found in all genera of the Nepenthales and rarely outside this group of plants, though its specific function in carnivory in these species is yet to be fully determined (Schlauer et al., 2005).
Anti-adhesive wax production used in prey capture
Epicuticular wax crystals are widespread in the plant kingdom and fulfil multiple functions. Within carnivorous plants, formation of wax is present in pitcher-forming plants: Catopsis berteroniana, Brocchinia reducta, Cephalotus, Sarracenia, Darlingtonia, Heliamphora and Nepenthes spp. (Riedel et al., 2003; Gaume et al., 2004; Gorb et al., 2005; Poppinga et al., 2010). However, the specific structure and composition of the wax has only been documented in Nepenthes spp.
In pitcher plants of Nepenthes spp., the inner surface of the trap is densely covered with epicuticular wax crystals (Riedel et al., 2003). This wax impedes insect adhesion, reducing their ability to escape from the pitcher. The wax constituents are secondary metabolites from primary alcohols, fatty acids, alkyl esters and n-alkanes, with the exact composition determining the specific formation pattern of the crystals. Triacontanal is the crucial compound in the formation of the wax crystals. This aldehyde homologue contributes 43 % of the entire wax crystal mass (Riedel et al., 2003). Triacontanal is common in a variety of non-carnivorous flora cuticular waxes and epicuticular wax platelets (Naeem et al., 2012). The other components of Nepenthes spp. pitcher wax are currently unknown. It is, however, likely that novel wax-forming metabolites are distributed throughout the crystal network or segregate into individual platelets and also form wax crystals to enhance prey capture (Gorb et al., 2005).
Mucilage and glue production for prey capture
Two variants of the sticky substance used in flypaper traps are produced by carnivorous plants. Within the Nepenthales (Drosera, Drosophyllum, Triphyophyllum), and within the Lamiales (Pinguicula and Byblis), they produce a polysaccharide-based mucilage. The mucilage of Drosera is composed mostly of primary metabolites and hence not included in Supplementary Data Table S1. myo-Inositol represents a significant proportion of the non-polysaccharide organic compounds, though is also a primary metabolite, derived from glucose (Loewus and Murthy, 2000; Kokubun, 2017). Though no detailed analyses are available, the other mucilage-producing carnivorous plants likely produce a similar product to the polysaccharide-based mucilage described. The mucilage is similar in appearance and characteristics, though confirmation of its chemical constituents would provide additional insight into the evolution of strategies to capture prey as the flypaper strategy spans at least five separate lineages [labelled (a) in Fig. 1; Pereira et al., 2012].
The second type of flypaper glue is not mucilage, nor an aqueous solution of acidic polysaccharides as described above; it is a lipophilic resin with high viscosity and is produced by species of Roridula. The resinous glue is composed of triterpenoids and acylglycerides (Simoneit et al., 2008). No saccharides or proteins have been previously detected within this glue, but traces of flavonoids have been identified in two species (Wollenweber, 2007). This glue is found to be considerably more viscous than the mucilaginous secretions of other carnivorous plants. In addition, this resin-based glue is much more resilient to water submergence and does not wash away as does the mucilage of Drosera spp. Here, two morphologically similar strategies to capture prey (flypaper) have evolved with distinctly contrasting chemical compositions to act as a glue: one is of a saccharide-based (primary metabolite) substance and one is of a resin-based (secondary metabolite) glue to achieve effective prey capture.
Prey capture signalling
Carnivorous plants have evolved strategies to detect prey capture and respond by synthesizing specific metabolites to advance the process of nutrient uptake from prey (Nakamura et al., 2013; Krausko et al., 2017). These signals may instigate trap movement, the accumulation of digestive enzymes, prey digestion and prevention of microbial decay of prey (Table 1).
Jasmonates are usually a component of plant defence systems. In Droseraceae, however, they have been co-opted to control the signalling of prey capture to instigate trap movement (Krausko et al., 2017). Jasmonates are lipid-based metabolites that typically act as phytohormones known to be involved in a wide range of regulatory processes. In particular, plant responses to stress, including herbivory and other kinds of biotic and abiotic stress, elicit jasmonate production, though they also serve as regulators of growth, photosynthesis and reproductive development (Wasternack and Hause, 2013). Accumulation of these metabolites is usually a fast response, occurring within minutes or hours of wounding or herbivory (Wasternack and Hause, 2013).
The sundew Drosera capensis increases concentrations of jasmonic acid and its conjugates in response to prey capture (Krausko et al., 2017). These increasing concentrations of jasmonic acid instigate leaf bending, which is targeted, due to jasmonate accumulation being controlled at an individual cell level, preventing a whole-plant response to jasmonates (Nakamura et al., 2013). This ensures that the traps curl and envelop the prey, increasing the trap surface area important in prey retention, digestion and assimilation. Drosera capensis has also retained the herbivory defence response instigated by levels of jasmonic acid. Drosera capensis therefore differentiates between prey capture and herbivory through propagation of electrical signals and consequent jasmonic acid accumulation (Krausko et al., 2017).
SECONDARY METABOLITES INVOLVED IN THE DIGESTION OF PREY
Once captured, the prey of carnivorous plants is digested. Enzymes are fundamental to the digestion of prey and identification of these specialized proteins is a current and ongoing endeavour (for a review see Ravee et al., 2018). Secondary metabolites can instigate enzyme production, enhance the efficiency of digestion and protect the plant from infection by preserving prey whilst it is being digested.
Three species that share the same carnivorous ancestor – Nepenthes alata and the two closely related species, Drosera capensis and Dionaea muscipula – demonstrate how the same secondary metabolites (jasmonates) can act as a biochemical signal for indicating the same stimuli but instigating a range of processes. Drosera spp. and Dionaea muscipula share a common Drosera-like ancestor (Gibson and Waller, 2009; Givnish, 2015), but they also have different prey-trapping mechanisms. Drosera spp. use sticky mucilage and slow leaf movement; Dionaea muscipula uses fast snap-traps (Forterre et al., 2005; Erni et al., 2008). Prey capture results in the accumulation of jasmonic acid, 12-oxo phytodienoic acid and isoleucine conjugate of jasmonic acid in all three species, Nepenthes alata, Drosera capensis and Dionaea muscipula. While jasmonates instigate enzyme secretion in all three species, it also initiates trap movement (to slowly envelop prey) in D. capensis (Mithöfer et al., 2014; Krausko et al., 2017) and in D. muscipula the accumulation of these secondary metabolites also instigates sealing of the ‘outer stomach’ (Libiaková et al., 2014; Pavlovič et al., 2017). In D. muscipula, jasmonates instigate production of the endopeptidase dionain, a compound involved in the chemical breakdown of prey (Libiaková et al., 2014). Trap movement in D. muscipula is, similarly to Drosera spp., instigated by intercellular electrical signals but relies on mechanical snap-buckling instability for the initial prey capture, followed by slow trap closure to form an ‘outer stomach’ instigated by phytohormones. Reliance on a physical snap-buckling mechanism over phytohormone-controlled leaf movement in D. muscipula is presumably because of the faster reaction times required for a snap-trap compared with a sticky flypaper trap for prey capture and retention. It seems likely that the prey-capture signalling function is common to the three species because of their shared carnivorous ancestor, but the function of this signal has somewhat diverged because a different trap strategy evolved in D. muscipula and Nepenthes spp.
Two additional groups of secondary metabolites appear to be part of the process of prey digestion: naphthoquinones and phenolics. In non-carnivorous plant species these secondary metabolites act as antibacterial protection against pathogenic attack on plant tissue (Krolicka et al., 2008). In carnivorous plants they appear to have been co-opted to preserve and aid in the digestion of prey by preventing decay from bacteria as well as functioning to protect the plant tissue during the process of prey breakdown.
Naphthoquinones are a class of organic compounds derived from naphthalene. In non-carnivorous plants, the naphthoquinone plumbagin is used as a deterrent against herbivorous insects, and has fungicidal and microbicidal effects (de Paiva et al., 2003). Similarly, juglone is inhibitory to insects, and so prevents herbivory. Plumbagin and juglone accumulate in the trap leaves of Drosera spp. in response to prey capture, suggesting that they play a role in the process of carnivory (Egan and Van Der Kooy, 2012). While their specific function has not yet been tested in carnivorous plants in situ, it seems likely that they enhance prey digestion by protecting the plant from pathogens that may otherwise infect the plant as a result of decaying prey. Nepenthes khasiana utilizes naphthoquinones as a chitin-induced antifungal agent found in its specialized pitcher fluid (Eilenberg et al., 2010). It appears to be used to preserve caught prey for digestion and assimilation of nutrients. Droserone and 5-O-methyldroserone have also been identified specifically for antifungal abilities in Drosera spp., D. muscipula and Nepenthes spp. (Table 1, Acton, 2012).
Presence of compounds involved in digestion within the same carnivorous lineage
Plumbagin is present in the trap tissue of every genus of the Nepenthales – Aldrovanda, Drosophyllum, Triphyophyllum, Dionaea, Drosera and Nepenthes – which share a common carnivorous ancestor (Table 1). Plumbagin is considered to function as an antimicrobial agent during digestion (Aung et al., 2002). Other possible roles, however, have been suggested. Plumbagin may function as an allelopathic, insecticidal, molluscidal antifeedant, while it also potentially may be important in attraction as a method to differentiate prey with pollinators (Krolicka et al., 2008; Raj et al., 2011).
It is suggested that, as the potential benefits of carnivory increase, the morphology of carnivorous plant traps becomes more complex (Ellison and Adamec, 2018). Secondary metabolites involved in digestion seem, however, to be fairly consistent across several different trap types. If plumbagin and its isomers are critical in protecting the plant during digestion or enhancing prey digestion, it would explain why these compounds have persisted regardless of trap morphology. Their presence across other taxa has, in this case, been investigated; 7-methyl-juglone and plumbagin are not present in five other lineages of carnivory [Cephalotus, Byblis, Roridula, Sarracenia and Heliamphora (of Ericales lineage) and Utricularia] (Zenk et al., 1969; Culham and Gornall 1994). These findings contribute important extensions to confirmation of compounds found in the Nepenthales. If preservation of prey and protection of trap tissue during prey digestion are such important adaptations, to the extent that they have persisted in all Nepenthales, why are these compounds not present across all carnivorous lineages? Other carnivorous lineages may have evolved novel compounds for the same function as the 1,4-naphthoquinones, such as plumbagin in Nepenthales, but to date this has not been investigated.
SECONDARY METABOLITES INVOLVED IN NUTRIENT ASSIMILATION AND WHOLE-PLANT RESPONSES
Currently, the metabolic role of and responses to assimilation of nutrients from prey are vastly unexplored. Carnivorous plants balance nutrient uptake and rate of photosynthesis within the same tissue (leaves), though root nutrient uptake is maintained in some species (Gao et al., 2015). This suggests, for some species, an interesting leaf–root signalling mechanism for nutrient status, distinct from typical nutrient dynamics in plants. Uptake of nutrients from prey via leaves has been shown to either maintain or increase root activity, the reverse response of leaf nitrogen uptake via stomata in non-carnivorous flora (Adamec, 2002; Gao et al., 2015). This strongly suggests a novel role of plant signalling in this complex nutrient regulation system.
Other aspects of the assimilation of nutrients from prey are known. For example, breakdown of non-absorbable compounds by secretion of enzymes in specialized glands and transmembrane transport of nutrients can occur through use of membrane carriers and endocytosis (Schulze et al., 1999; Plachno et al., 2006; Adlassnig et al., 2012). Pulse–chase experiments and research into metabolites that are taken up by the plant via prey digestion have confirmed the uptake of carbon and nitrogenous compounds, but identification of subsequent plant response has varied (Adamec, 2002; Rischer et al., 2002; Karagatzides et al., 2009). Carbon from prey-derived protein in Nepenthes insignis was found to be utilized for production of plumbagin (Rischer et al., 2002). Conversely, Drosera capensis was found to have similar levels of quercetin and kaempferol before and after prey capture (Kováčik et al., 2012). Plant response and signalling using plant-synthesized metabolites in response to the assimilation of animal metabolites remain largely unexplored. Metabolic profiling of trap, non-carnivorous leaf and root tissue before, during and after nutrient uptake from prey may provide insight into any biochemical signalling within the plant to control assimilation or regulate nutrient balance within the whole plant. Research in this area may provide insight into exaptation of current plant regulation processes or a novel method for plants to control nutrient uptake via leaves.
OCCURRENCE OF SECONDARY PLANT METABOLITES INVOLVED IN CARNIVORY ACROSS PLANT TAXA
This review has identified ~170 secondary plant metabolites that have, or are highly likely to have, a role in some aspect of the carnivorous habit, some of which are isomers or derivatives or select groups of compounds. Of these, 48 secondary metabolites have been found to play a role in carnivory in more than one genus (Table 1; grey bars in Supplementary Data Table S1). Twenty-six compounds are shared across independent evolutionary lineages of plant carnivory (Nepenthales and Sarraceniaceae; unboxed ticks in Table 1). However, only Nepenthales and Ericales have been investigated for secondary plant metabolites from an ecological function perspective (Fig. 1), and of the 33 studies used for the generation of Supplementary Data Table S1, only one examines the metabolic role in carnivory across multiple evolutionary lineages (Jürgens et al., 2009). There are, however, many studies identifying these and other compounds across other taxa for some kind of anthropogenic use (pharmaceuticals or biotechnology) or as taxonomic markers, but their plant function is not specified (e.g. Budzianowski, 1996; Muhammad et al., 2013). We excluded these studies from this review because these artificially derived compounds may have no function in the carnivorous habit.
The origins of the evolution of carnivory are beginning to be determined, but there remain key components of the evolution of the carnivorous syndrome that are yet to be identified. Aspects of the evolution of carnivory have recently been linked to plant defence (Pavlovič and Saganová, 2015). This link is appropriate given the similarity in function of response to and digestion of prey compared with responses to herbivory using antimicrobial and insecticidal compounds and phytohormones. Similarly, most metabolites used for attraction are related to flower or fruiting scents (Kreuzwieser et al., 2014; Jaffé et al., 1995). Given that carnivorous plants, so far, are all angiosperms, it would be interesting to investigate the genetic origins of these compounds, as it is currently unclear whether compounds for attraction are similarly co-opted from pollinating and dispersal mechanisms, or whether novel co-evolution has taken place to mimic olfactory cues.
The majority of metabolites, and metabolites identified so far across different taxa, are involved in the attraction and or capture phases of plant carnivory; this may, however, be falsely disproportionate (Supplementary Data Table S1). Over-representation of metabolites involved in attraction for plant carnivory is understandable, given the relative simplicity of inferring their functional role. Presence of volatiles found on traps is logically assumed to have some olfactory attraction for animals and up- or downregulation of these compounds following prey capture reinforces this assumption. Similarly, composition of sticky mucilage exuded on traps leads to a conclusion of a function in capture or retention (though potentially also attraction). Only a handful of studies identifying compounds in attraction or capture specifically test these compounds with prey capture assays (e.g. Jürgens et al., 2009). Furthermore, classification of many VOCs is from a technical chemical standpoint and may not have a significant impact on olfactory attraction in situ as their volatility is not realized under biologically relevant temperatures. More stringent restrictions of inclusion of these compounds would reduce the overall number of compounds significantly, though perhaps unnecessarily. Further investigation of these compounds is required to determine their function, rather than their outright exclusion as important compounds. This, coupled with the challenge of identifying a carnivorous role of a compound in digestion and assimilation, may have led to an over-representation of certain compounds in the literature and may erroneously suggest that only these aspects are strongly reliant on secondary plant metabolites. There are currently many secondary metabolites extracted from specifically carnivorous plants for pharmaceutical, pseudo-medicinal or biotechnological use (Ellison and Adamec, 2018, and references therein). These could be compared with chemicals where a functional role is known in order to establish metabolic similarities across taxa. Experiments testing the function of these known compounds would be an effective way to rapidly grow the library and taxonomic presence of compounds strictly related to plant carnivory that function in processes other than attraction or capture.
CONCLUDING REMARKS AND FUTURE PERSPECTIVES
Secondary metabolites are known to be important to carnivorous plants in the attraction, capture and digestion of prey for nutrient assimilation. There is a high degree of co-option as well as generation of novel metabolites to aid the carnivorous syndrome. This is likely due to inherently high chemical diversity and the utility of these compounds for multiple functions (Moore et al., 2014). A key question is the importance of secondary metabolites in carnivorous plant evolution. Have similar metabolic approaches to carnivory evolved independently in different carnivorous plant lineages? There is evidence of metabolites involved in carnivory present in more than one genus and, in some cases, these are found across independently evolved lineages (unboxed ticks in Table 1; also highlighted dark grey in Supplementary Data Table S1). There may be great potential in examining the role of novel secondary metabolites for carnivory in different species that differ in their morphological approach to carnivory but share a common ancestor (e.g. Nepenthales in purple block in Fig. 1 and dark grey block in Table 1).
There is growing interest in the role of secondary metabolites in the rapid diversification and evolution of plants to new environments. This is due to high chemical diversity, with rapid generation of novel compounds being attributed to adaptation rather than mutation (Moore et al., 2014). Carnivorous plants have been found to have huge chemical diversity, most of which we know about through their use in biotechnology (Ellison and Adamec, 2018, and references therein). We hypothesize that metabolite diversity provided a mechanism for the evolution of carnivory in plants, and that this continued diversity facilitates rapid evolution into new environments. Due to the multiple times carnivory has independently evolved and the general restriction of carnivorous plants to high-stress environments, these plants are an ideal system to investigate whether metabolic diversity may have been or is a way for novel traits to evolve and be maintained. Metabolites are clearly integral to the survival of carnivorous plants in nutrient-deficient environments and further investigation of the carnivorous habit will continue to provide new insights into novel pathways of plant evolution.
SUPPLEMENTARY DATA
Supplementary data are available online at https://dbpia.nl.go.kr/aob and consist of the following.
Table S1: secondary plant metabolites involved in plant carnivory.
Funding
This work was supported by a Loughborough University PhD studentship to C.R.H.
ACKNOWLEDGEMENTS
We thank Richard Harland for his help with chemical groupings and Angus Davidson for assistance with plant drawings. We also thank the reviewers for their useful comments.